Yunyun Gao, Johannes Kaub, Gianluca Santoni, Nicholas Pearce & Andrea
Thorn
This blog post was published in Crystallography Reviews, please cite: https://doi.org/10.1080/0889311X.2023.2222275
Abstract
The SARS-CoV-1/SARS-CoV-2 main protease cleaves the nascent viral polyproteins into biologically functional molecules, which are essential for viral reproduction inside the host cell. With more than 500 crystal structures available, it is one of the most heavily researched coronavirus proteins and a popular drug target. This review focuses on putting the function and structure of the main protease into a historical perspective, highlighting the structure-based design of inhibitors of the main protease and discussing potential future research directions.
1. Introduction
Main protease (often called MPro or Mpro) is one of the most important non-structural coronavirus proteins, responsible for cutting viral polyproteins into functional units, and thus essential for the infection cycle. Main protease cleaves major parts of coronavirus polyproteins (pp1a and pp1ab) at eleven conserved sites [1,2], producing fully functional proteins which ultimately allow the virus to hijack the host cell and facilitate viral amplification [3].Main protease is the fifth non-structural protein (nsp) on both pp1a and pp1ab and is therefore also known as nsp5 [2]. Inhibition of main protease can effectively prevent coronavirus replication, making it an ideal target for drug development [4,5]. While this review focuses on severe acute respiratory syndrome coronavirus 2 (SARS-CoV-2) as well as severe acute respiratory syndrome coronavirus (SARS-CoV-1), the main protease is present in all known coronaviridae. Main protease is a cysteine protease characterized by a conserved cysteine-histidine catalytic dyad and is therefore also referred to as the 3C-like protease (3CLpro) due to the similarity in the cleavage-site specificity to that of picornavirus ‘3-Chymotrypsin’ protease [1]. Experimental investigations into main proteases of enveloped, positive-stranded RNA coronaviruses were able to reveal many features even before the first molecular structure was solved. Firstly, the catalytic residues are affixed in two chymotrypsin-like β-domains in a form of dyad or triad [3,6,7]. Secondly, the specificity of the protease is modulated by the substrate sequence around the cleavage site. For a given substrate P with a sequence of . . . P2–P1↓P1–P2 . . . (where ↓ denotes the cleavage site), the most important sites are P1, P2 and P1. For cleavage by main protease, the favoured sequence is (Leu/Met/Phe)–Gln↓(Ala/Ser/Gly) [8,9]. Thirdly, the C-terminal region has an extension of 110 amino acid residues – unique compared to other known prototypic 3C proteases – which is required for proteolytic activity [10]. Nevertheless, in the absence of a structural model, the precise mechanism of the proteolytic activity could only be speculated. The first available structure of a coronavirus main protease, from the transmissible gastroenteritis virus (TGEV), was solved using selenomethionine-based multiwavelength anomalous dispersion (MAD) and reported in 2002 [11]. This crystal structure confirmed that the catalytic centre is a dyad with a cysteine acting as the nucleophile and a histidine as the general acid/base. The protomer folds into three domains of which domain I and domain II form the chymotrypsin-like antiparallel β-barrels and the C-terminal extension forms a five-α-helical domain III. Two protomers assemble into an obligate homodimer by the intermolecular interactions between the interfacial residues of domains II and III and the N-terminus residues of the other monomer. The structural arrangement also provided evidence that the autocatalytic cleavage occurs with the cleaved bond in a trans conformation [11]. Themost studied coronavirus soon became SARS-CoV-1 (severe acute respiratory syndrome coronavirus) as it spread rapidly during the SARS outbreak in 2002 and 2003 [12–14]. This virus, a member of the coronavirus subfamily sarbecoviruses (severe acute respiratory syndrome-related coronaviruses) has a main protease with sequence identities of around 50% compared to those in other prominent members of the coronavirus family such as human coronavirus 229E (HCoV-229E) and Middle East respiratory syndrome coronavirus (MERS-CoV). This indicates highly conserved tertiary and quaternary structures [1,11,15,16]. Due to the prior knowledge of coronavirus gene expression and withmultiplemodels available formolecular replacement, structures of SARS-CoV-1main protease were quickly determined: the first crystal structure was available just two months after the sequencing of the viral RNA[15,17]. The SARS-CoV-1main protease shares common structural features with other previously solved coronavirusmain protease structures, such as dimerization and the substrate-specific active sites. This similaritymade inhibitors of other coronavirusmain proteases potential drug candidates for SARS [1], but substratebound structures of the SARS-CoV-1 main protease revealed that the catalytic site varies around the catalytic dyad, affecting the site specificity of the substrate [15,18,19]. While the outbreak of SARS was successfully controlled by public healthcare campaigns, reemergence of a coronavirus pathogen for humans was still considered a potential risk with the identification of new strains including human coronavirus NL63 (HCoV-NL63), human coronavirus HKU1 (HCoV-HKU1) and MERS-CoV [20,21]. Over the following years, crystallographic SARS-CoV-1 research focussed on twomajor efforts: to better understand the enzymatic reaction mechanism, especially the dimerization [22–27], and to enable structure-based drug design [18,23,24,28–37].Thewealth of knowledge derived fromthese studies later provided a head start in understanding the main protease of a new virus, SARS-CoV-2. The genome for the novel coronavirus SARS-CoV-2 (severe acute respiratory syndrome coronavirus 2) was published on January 25th 2020 [38]. The virus and the associated disease, COVID-19, spread across the world within months and caused a global pandemic, which continues to impact almost all aspects of society. In the campaign against COVID-19, the SARS-CoV-2 main protease was once more given considerable attention due to its small size, the amount of existing research, and important role in the infection cycle. The main proteases of SARS-CoV-1 and SARS-CoV-2 have a sequence identity of ∼96%, with the 12 amino acid differences distributed across residues distant from the active site [39]. The first SARS-CoV-2 crystal structure was solved swiftly, within three weeks of the deposition of the viral RNA sequence [16], using previously solved main protease structures as homologous models. In the following two years, more than 400 crystal structures of the SARS-CoV-2 main protease have been deposited in the PDB, including several large-scale efforts: a fragment screen conducted at Diamond Light Source (UK) [4], a drug molecule screen at Deutsches Elektronen- Synchrotron (DESY; Germany) [5] and most recently a compound optimization screen at the MAX IV Laboratory (Sweden) [40]. Various studies have used the available structures to identify/develop/optimize potential inhibitors, to elucidate enzyme mechanisms, to recognize the binding mode of repurposed drugs and as a target for method research (Figure 1).
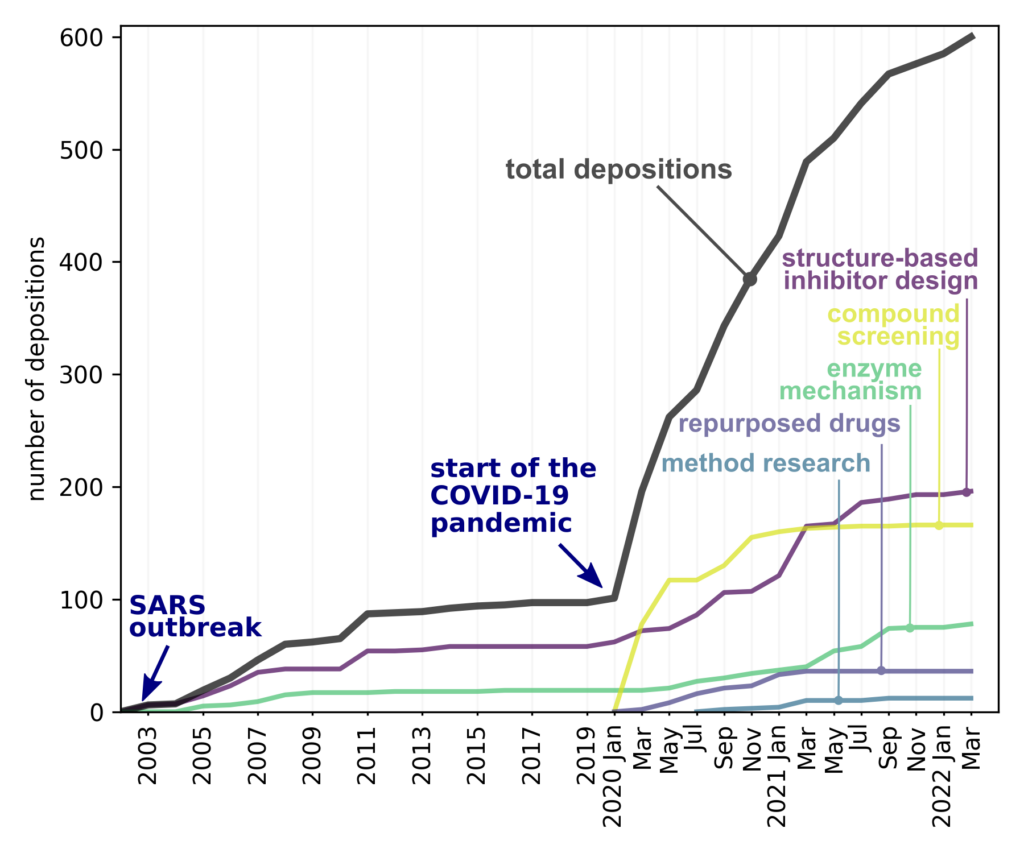
Figure 1. Combined PDB depositions of SARS-CoV-1 and SARS-CoV-2 structures over time. Lines indicate the depositions cited by published studies focusing on different topics: structure-based inhibitor design, enzyme mechanism, drug repurposing and methods research. The number of total depositions includes additional structures without associated publications. Data from September 2022.
2. Structural overview
Coronavirus main protease molecules are readily crystallizable and almost all available structures have been solved using X-ray crystallography (712 total depositions in the PDB as of Sep. 2022: 705 X-ray structures, two NMR structures, four joint neutron/X-ray structures, and one electron microscopy structure). 93% of the deposited sarbecovirus main protease crystal structures have a high-resolution limit better than 2.5 Å, and 64% are better than 2.0 Å. The highest resolution structure is 7k3t, a free apo formdetermined to 1.2 Å resolution. 6lu7 [16] is the most cited structure and a popularmodel formolecular docking studies [41]. In sarbecoviruses, the mature catalytically active form of the protease forms obligate homodimers (Figure 2(a)), with each monomer comprising 306 amino acid residues. In each protomer (Figure 2(b)), domain I (residues 8 to 101) and domain II (residues 102 to 184) are fairly rigid [1,11]. The active dyad Cys145–His41 is embedded in a pocket formed by the domain I and domain II barrels. N-terminus residues 1–7 are a remnant of the autoproteolytic process, and often called the ‘N-finger’. One of these residues, Ser1∗ (residues from the neighbouring protomer will be indicated in the rest of the text as e.g. Ser1∗), forms interactions with conserved residues in the other protomer, making a salt bridge to the side chain of Glu166 and backbone interactions with Phe140. These interactions structurally ‘cap’ the outside surface of the active site. Domain III (residues 200 to 302), also known as C-terminal domain, is connected to domain II by an interdomain loop (residues 177 to 199) which links β-strand 12 and the α-helix 3. Domain III is more flexible compared to the N-terminal domains [42–44], and though distant from the active site, domain III is functionally important as it is indispensable for enzyme dimerization [22,45].
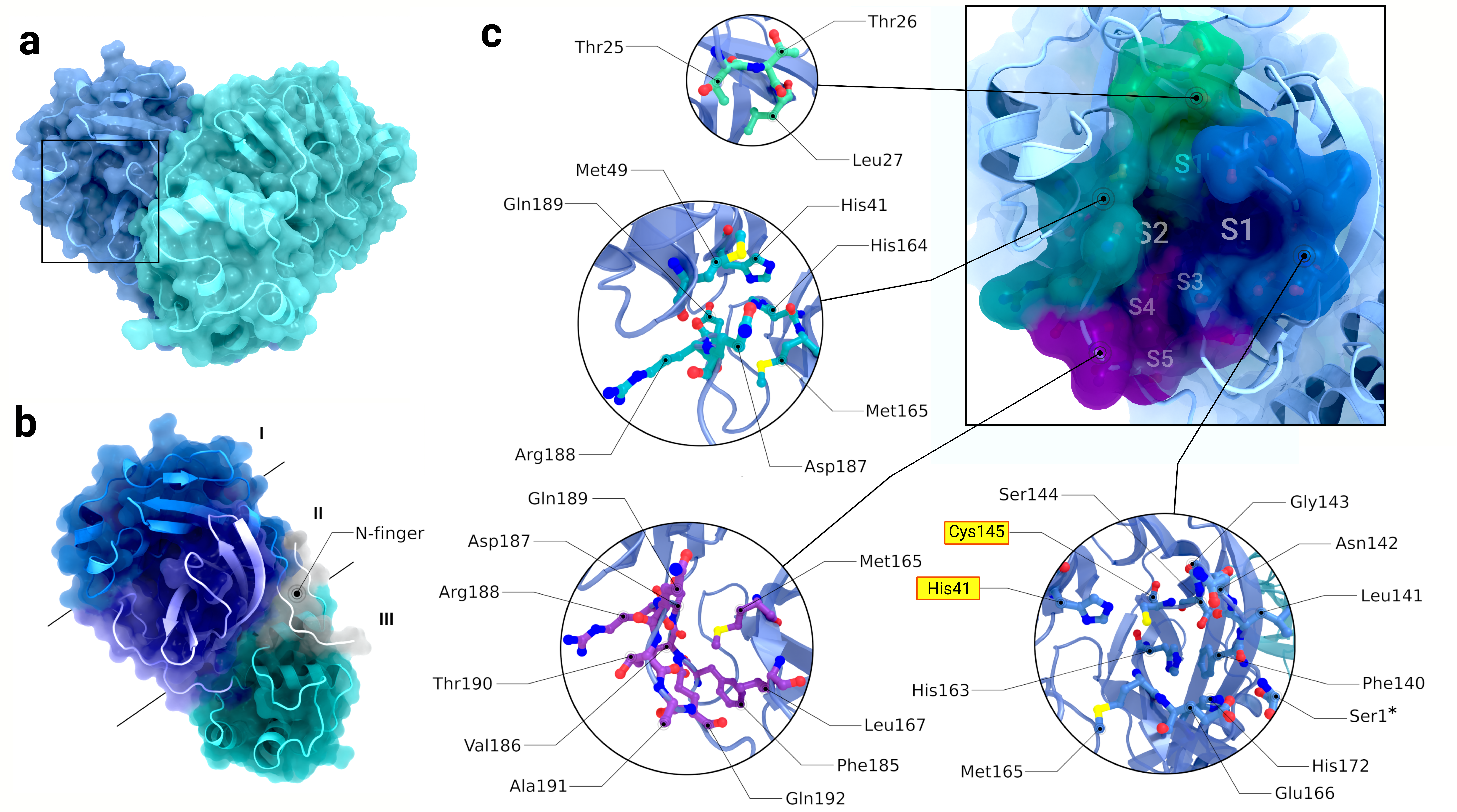
Figure 2. An overview of the structure of the SARS-CoV-2 main protease (PDB ID: 6LU7). (a) Surface and cartoon representation of the homodimer in the free apo form with each monomer in a different colour. (b) Domain structure of the main protease monomer: domain I, domain II, domain III and the N-finger are indicated (blue, purple, cyan and grey, respectively). (c) Introspection of the binding cleft and active site. S1 subsite is highlighted in blue, S2 in cyan, S1 in green and S3 to S5 in purple. Pull-outs: views of subsites with component residues labelled. The Cys145–His41 catalytic dyad is highlighted yellow. Molecular surface representations were rendered using Protein Imager [173]. Creator: Coronavirus Structural Task Force - Yunyun Gao, License: cc-by-sa
2.1. The active site
The Cys145–His41 active dyad is conserved across all coronaviruses [46]. Both kinetic studies [47] and the following molecular dynamics simulation [48] suggest that Cys145 and His41 adopt an uncharged rest state in the free apo form. An interpretation of neutron data of a SARS-CoV-2 main protease crystal at pH 7.0 and room temperature (PDB ID: 7jun) shows that His41 is positively charged through protonation of both nitrogen atoms of the imidazole side chain and that Cys145 is deprotonated and negatively charged [49]. It has also been indicated that Cys145 is readily oxidized to sulfinic acid [11,50], but such oxidation could have been triggered by incident X-ray radiation, especially for those structures determined at room temperature [50]. For sarbecoviruses, the distance between the thioester S of Cys145 and the imidazole N of His41 is in the range of 3.6 to 3.7 Å; this distance is beyond the length for a typical hydrogen bond between the thiol group and the histidine nitrogen. A catalytic water is trapped in a pocket formed by His164, Asp187, Arg40 and His41, which likely plays an important role in holding the active site in the proper geometry [44,51]. This water molecule forms hydrogen bonds to the N atom of His41, the Oδ atom of Asp187 and the Nδ atom of His164 [1,49,52]. Two residues in this pocket – Arg40 and Asp187 – forma salt bridge and are absolutely conserved [51], whereasHis164 is not absolutely conserved and the H164Lmutation generates no significant effect on the proteolytic activity [11]. The specificity of substrate binding is provided by several subsite pockets around the active dyad, whose names derive from the substrate residue that occupies them, i.e. S3, S2, S1 and S1 (the P1 residue binds in subsite S1).
2.2. The S1 subsite
The S1 subsite is a hydrophilic pocket occupied by a glutamine residue for all cleavage sites on the main protease substrates (polyproteins pp1a and pp1ab) [13,38], which implies the significance of this site for substrate recognition. An oxyanion hole is formed by the loop comprising residues 140–144 and the cap described above formed by Ser1∗, Glu166 and Phe140 (Figure 2(c); bottom right). Such an arrangement is a key component of the catalytic machinery that stabilizes a transition state oxyanion during substrate hydrolysis [53]. The main chain atoms ofMet165 and the two side chains of His163 and His172 form the back wall of the pocket. In all the reported monomer structures, the S1 subsite loses the specificity and the oxyanion hole also completely collapses [22,25,54]. However, while Ser1∗ may be important for substrate recognition, truncating this residue does not lead to a complete loss of enzymatic activity (see dimerization section below).
2.3. The S2 subsite
The S2 site is a wide hydrophobic pocket, and although it has a high specificity for Leu, it can also accommodate Phe and Met residues [44]. The subsite is bordered by Asp187, Arg188, Gln189 and His164 and capped by the side chains of His41, Met49 and Met165 (Figure 2(c); centre left). The side chains ofMet49,Met165 andGln189 have been proposed to be flexible to adapt to the presence of various P2 groups [5,16,51,55]. For SARS-CoV-1, it was previously reported that a S2–S3 cooperative binding mode exists (S3 subsite residues: Thr25, His41, Cys44–Ala46, Met49). Cooperative binding would mean that for the substrate residues flanking the cleavage sites in SARS-CoV-1 pp1a/pp1ab, a Phe residue at P3 is exclusively required whenever the S2 subsite is occupied by a Phe residue and the S2-Phe binding conformation appears [27]. This mechanism seems not always to be relevant in SARS-CoV-2: for example, in the apo structure, the S1–S3 pockets are similar to those in the acyl-enzyme intermediate structure (PDB ID: 7kph) [51], which indicates the formation of S3 is not dependent on the binding of P2.However, a docking study using the acyl-enzyme intermediate structure shows that a highly active non-covalent inhibitor, 17a [36], occupies the S2 and S3 subsites with two phenyl-like groups at the same time [51], suggesting that the S2–S3 cooperative bindingmodemay be important for designing a high-affinity inhibitor. It is also suggested that when designing an inhibitor targeting S2–S3 cooperative binding, a structure with the S2–Phe binding conformation such as 7jkv could be considered [56].
2.4. Other subsites
Other subsites are generally regarded as less specific to certain amino acids. The S3 subsite has no well-defined pocket [57] but can be characterized by the backbone of Glu166: the interactions between the Glu166 backbone and the P2/P3 amide carbonyl along with the P3/P4 amide hydrogen are conserved for physiological substrates [27,51,58]. The S1 subsite is a shallow pocket in proximity to the catalytic dyad surrounded by Thr25, Thr26, Leu27 and Gly143 (Figure 2(c); top left). The S2 subsite is a narrow but deep pocket composed of residues Thr26, Asn28, Tyr118, Asn119 and Gly143. While the S1 site exhibits specificity to small side groups such as Ser, Ala and Gly, crystallographic snapshots from the natural substrate-bound structures suggest that the P1 side chain is not fully sterically matched to the S1 pockets; the same observation applies to P2 and P3 [24,51]. The S4 and S5 subsites are exposed to the solvent and structurally less defined (Figure 2(c); bottom left). The S4 subsite is a deep hydrophobic pocket formed by the loop consisting of residues 185–192 and the side chains of Met165 and Leu167. Although the binding specificity is less pronounced for these subsites, they are important for lead optimization:modifications targeting S1 and S4 have been structurally evidenced to be effective [57,59–61].
2.5. Dimerization
The dimer interface includes the N-finger, α-helix 1 (residues 10 to 15), β-strand 9 (residues 121 to 129), residues 132–142 and α-helix 7 (Arg 298, Gln299) (Figure 3). Sequence conservation is particularly pronounced for the N-finger and loop residues 137–142 across all the coronavirus main proteases [11,62]. The tip of the N-finger is also a component of the S1 subsite.Mutagenesis studies [63] show that truncation of residues 1–3 results in a loss of enzyme activity by 24%, despite preservationof the dimer.However, once the truncation reaches conservedArg4, themain protease dissociates into amonomer form with little to no activity.The side chain ofArg4 reaches into the domain II–domain III interface of the other protomer and forms hydrogen bondswith Lys137∗ (highly conserved) and Glu290∗ (completely conserved in known coronaviruses). The importance of these interactions is confirmed by the complete loss of activity and dimerization in an E290Amutant [64]. Molecules with a domain III deletion also do not form dimers [65–67]. The highly conserved α-helix 1 is another key contributor to dimerization, as the hydrogen bonds formed by Ser10–Ser10∗, Gly11–Glu14∗ and Glu14–Gly11∗ act as an anchor to fix the Nfingers of the two monomers [63,65] and S10A, G11A [54] and E14A [68]mutants all form predominantly monomers with no activity. Gln299 is completely conserved among coronavirus main proteases and the side chain of Gln299 hydrogen bonds the main chain of Arg4, including those in the three monomeric structures [22,25]. Additional hydrogen-bonding residues Ser123–Arg298∗, Ser139–Gln299∗ and their inverses are also conserved [52,69]. Interestingly, S123A and S139A mutants lead to very limited dissociation and retain substantial enzyme activity [25,67,70], while the R298A and Q299A mutants result in an activity of ∼1% compared to the wild-type enzyme [22,70]. A Gln299 to Glu, Lys, or Asp mutation reduces enzyme activity by more than 90%, but R298K has no significant effect on the enzyme activity [67,70]. In a structure of the immature protease (PDB ID: 7kfi), where the dimerization was interfered by a N-terminal insertion, the intermolecular interactions Ser123–Arg298∗ and Ser139–Gln299∗ do not exist while the hydrogen bonds – either intermolecular or intramolecular – related to the five absolutely conserved residues Arg4, Ser10, Gly11, Glu14 and Gln299 are intact. In the immature dimer, the N-finger Ser1 hydrogen bonds to Phe140∗, but does not fully cap the S1 subsite, leading to an incomplete oxyanion hole. In the active assay referring to this immature structure, the protease shows only 6% of the enzyme activity compared to the mature wild-type enzyme [71]. In the active dimer, domain III of the two protomers depart fromeach other. One crystal structure of domain III alone (PDB ID: 3ebn) was reported to form a dimer with swapped subdomains [66], in which the α-helix 3 (residues 200-214) of one domain III protomer is embedded in the other four helices from the other protomer. The crystal structure of a monomeric G11A mutant (PDB ID: 2pwx) shows a conformation that domain III bends toward the side of the N-finger, and was considered as evidence that the dimerization is initiated through the domain III association followed by separation in the mature protein [54].However, it is hard to imagine that such a domain swap is energetically favoured in the formation of the active dimer. Further studies postulate instead that dimerization might not be initiated by domain III association but rather by two key anchor components, Arg4 and α-helix 1. Then the intermolecular interactions between α-helix 7 and the serine cluster (Ser123, Ser139) brings two protomers into contact and secures Ser1∗ into a complete S1 subsite [18,51,54].While domain III may not initiate the dimerization, compounds binding to domain III do interrupt the form of dimer [72]. Essentially, these allosteric inhibitors disrupting the dimerization should warrant more consideration as novel drugs.
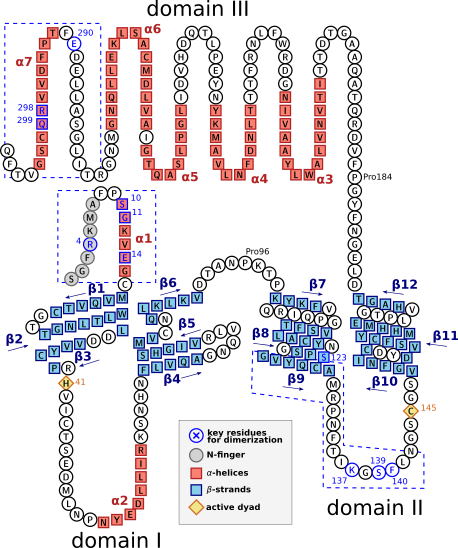
Figure 3. Structure-based sequence representation of the SARS-CoV-2 main protease. α-helices, β- strands and N-finger are marked as red squares, navy squares and grey circles. The Cys145–His41 active dyad is marked as yellow diamonds. Key residues for dimerization are highlighted in blue. Dashed boxes indicate regions associated with the dimer interface. Residue markers are created using Protter [174]. Creator: Coronavirus Structural Task Force - Yunyun Gao, License: cc-by-sa
2.6. Structural variability and flexibility
There has already been an extensive analysis of the differences between the large number of main protease structures [42]. Structural alignments of available structures indicate that the global differences between structures determined at room temperature and at cryogenic temperatures as well as using different radiation sources – various synchrotrons, X-ray free-electron lasers and in-house diffractometers – are not significant, and that the overall configuration of the two N-terminus domains is largely structurally invariant [42]. However, some of the conformational space ofmolecules is revealedbydifferences between the different crystal forms: for example, one crystal form shows distinct reorientations for the C-terminal domain, but it is unknownwhether this is biologically relevant or merely a conformation induced by crystal packing [42]. Comparisons between ligand-bound and apo protein structures under comparable physical conditions have revealed significant deformations of the active site, suggesting an expansion of the binding site upon substrate acquisition [51,52], though there is also considerable variability within sets of apo and ligand-bound structures [42], suggesting inherent flexibility rather than purely substrate-induced conformational changes. Although a number of residues surrounding the binding site are observed to rearrange upon binding, two sites are identified as particularly flexible in molecular dynamics simulations: the P2 helix and the P5 loop [52]. The flexibility of these elements is supported by ensemble refinements against the crystallographic data [73] aswell as byanewapproach for extracting molecular flexibility directly fromexperimental B-factors [74]. The latter analysis also hints towards heterogeneous behaviour of an external loop (residues 62-80), which exhibits varying levels of disorder in different crystal forms especially for lower-resolution structures [74], though once more this may only be a crystal-induced conformation. The C-terminus of the protein is confirmed as flexible and partially disordered in structures [42,51]; however, its proximity to the binding site raises the question of what this flexibility could mean for the role of the C-terminus in substrate binding. It has been suggested that differences between structures at different temperatures may make the more physiologically relevant structures preferable for secondary applications such as molecular docking into the binding site [52], though some of the identified differences were due to simple modelling errors [42]. Another study collected a series of crystals at different temperatures and showed that though the average atomic positions of the protein may be consistent across temperatures, the extent of variability changes significantly as the temperature is increased from cryogenic to near-physiological [73]. Although there may be some question as to the precise nature of the changes between temperatures, all of these studies agree on the highly flexible/plastic nature of the main protease binding site cleft.
3. Main protease inhibitors as therapeutics
Viral protease inhibitors have been developed as the antiviral agents for many infamousviruses, such as the human immunodeficiency virus (HIV) and the hepatitis C virus[75–77]. TheCOVID-19 pandemic and the structural conservation ofmain protease acrossrelated viruses make it and its homologs one of the most extensively studied antiviral drugtargets.The most eminent coronavirus main protease inhibitors were peptidomimetic compoundstargeting SARS-CoV-1 [1,15,31,33]. The peptide chain first interacts specificallyand non-covalently with the enzyme at the active (sub-)sites, bringing a warhead intothe catalytic site. The warhead group then attacks Cys145, forming a covalent bond andblocking the active site irreversibly. The mechanism of a number of peptidomimetic warheadinhibitors has been directly observed in crystal structures.Warhead groups observedin crystal structures include chloromethyl ketone [15], unsaturated ester [19,28,60,78],epoxide [29,79], aldehyde [32,33,44,55,57,78,80], nitrile [35], α-acyloxymethylketone [81]and various Michael acceptors [59,82–84]. Almost all potent peptidomimetic warheadinhibitors contain a 2-pyrrolidone (often referred as γ -lactam)moiety in the P1 position asa substitute of glutamine in the natural substrate.Utilized P2moieties include hydrophobicsubstitutes such as leucine and phenylalanine side chains, and cyclopropyl and cyclohexylgroups can be similarly accommodated [55,83]. The crystal structures of the main proteasein complex with inhibitors show that 2-pyrrolidone can form hydrogen bonds withHis163, Glu166, and Phe140 in the S1 subsite. Besides the P1 position, systematicmodificationson other subsites – such as P1 [28], P2/P3 [55,57,83,85] and P4/P5 [44,60,86] – havealso been investigated in order to improve the binding affinity. In addition to the substrateanalogues, a few warhead inhibitors are identified by drug repurposing [87–89]. Amongthese, one of the most prominent compounds is GC376 [87,89–92], a preclinical inhibitoragainst the main proteins of feline infectious peritonitis coronavirus.GC376 adapts a bisulfitewarhead and consists of a 2-pyrrolidone, a leucine and a benzyl group in the P1, P2and P3 position, respectively (Figure 4(c)). A number of subsequent lead optimizationstudies, with the evidence of specific binding modes, demonstrated an improved effectivenessof GC376 analogs against main protease by modification of its chemical structure[55,57,84,93,94].The peptide warhead inhibitors also led to the design of non-peptide inhibitors withlow molecular weight. Two early crystal structures in complex with non-peptide warheadinhibitors bearing benzotriazole ester [31] and halomethyl ketone moieties [95]were reported. Such research is limited, potentially due to the fact that these non-peptideinhibitors of shorter lengths did not show a significant increase in affinity compared to thepeptidomimetic ones [37,96].Recently, however, large screening campaigns have identifieda number of highly potent non-peptide covalent inhibitors, including ebselen derivatives[97] and myricetin derivatives [98].The development of non-covalent inhibitors is another important approach to overcomethe potential off-target side effects and toxicity that accompanies the irreversible bindingof covalent inhibitors [40,99]. Despite extensive virtual screening campaigns describinginhibitors that could bind to the main protease [100], only a few hits were validatedby the crystal structures of their complexes [30,34,36,61,101–105]. Among them, ML-300 [36] (Figure 4(h)), ML-188 [101] (Figure 4(i)) and perampanel [106] (Figure 4(j)) have been frequently used as the lead compounds for further optimization [61,102,107]. The potential scaffolds identified by virtual screening were diverse, but the experimentally determined complexes mostly reported hydrogen-bonding interactions between a pyridinyl-like or a chlorophenyl-like moiety on the inhibitor and the His163 side chain of the S1 subsite; this interaction is therefore thought to be key to achieving binding affinity in a non-covalent inhibitor [61,108,109]. There are also efforts to convert the identified noncovalent lead compound to more potent covalent analogues by combining the identified chemical features and a reactive warhead [110,111]. To date, the most potent non-covalent inhibitor with a known binding mode is s-217622 (Figure 4(k)), which was a structure-based optimization over a pharmacophore hit from a combination of virtual and biological screening [112]. s-217622, later named ensitrelvir, shows ideal drug metabolism and pharmacokinetic profiles and has a confirmed antiviral efficiency in phase 2a trials [113]. In addition to structure-based drug design efforts, the broad SARS-CoV-2 drug repurposing campaigns also generated attractive drug candidates against main protease. Multiple crystal structures of main protease in complex with different drugs – baicalein [114], carmofur [115], shikonin [116], leupeptin [5,117], narlaprevir [117,118], boceprevir [89,117,119,120], telaprevir [117,119,120], masitinib [121] and myricetin [98] – have been reported. Among these compounds, baicalein, shikonin and leupeptin are reported as reversible inhibitors since the binding modes involve only hydrogen-bonding interactions to the S1 and S2 subsites. Carmofur, an approved antineoplastic drug [122], binds covalently to the catalytic Cys145 residue and occupies the S2 subsite with the alkyl chain of the hexylcarbamoyl group.However, a study on the enzymatic assay and the binding assaywith the addition of the reducing reagent dithiothreitol suggest that shikonin and carmofur are in fact non-specificmain protease inhibitors [123]. The identification ofmyricetin provides pyrogallol as a warhead in the design of new inhibitors [98]. Narlaprevir, boceprevir and telaprevir are approved serine protease inhibitors against hepatitis C virus infection and are peptidomimetic warhead inhibitors, bearing α-ketoamide warheads but with different hydrophobic substituents. Narlaprevir, boceprevir and telaprevir complexes show similar conformational changes in the active subsites as observed in the other peptidomimetic inhibitors [51,117], suggesting oncemore that the active pocket is intrinsically flexible and able to adapt to different chemical groups by alternating the conformations of the subsites. Narlaprevir, boceprevir and telaprevir also showa certain binding specificity against SARSCoV-2 main protease [100,123,124], which makes them promising lead compounds for further structure-based optimization. Interestingly, advanced optimization based on variable warheads and the backbone structures of the three serine protease inhibitors and their binding modes have also been explored and yield a number of highly potent inhibitors, such as MI-23, MI-09 and MI-30 [119] as well as UAWJ9-36-1 and UAWJ9-36-3 [125] (Figure 4(e)). PF-07321332 (PF-332) [126–128] (Figure 4(f)), a novel reversible covalent peptidelike main protease inhibitor by Pfizer (nirmatrelvir; the oral antiviral drug Paxlovid is a tablet of nirmatrelvir+ritonavir), has confirmed efficacy from phase 2/3 trials [69]. Compared to the initial candidate PF-00835231 [59], several notable features were introduced: the nitrile warhead is selected based on the considerations of bioavailability and scale-up synthesis; the cyclic P2 moiety from boceprivir/MI-09 [119] was adapted to not only better fit the S2 subsite but also to improve the passive permeability by removing the hydrogen bond donor from the P2/P3 amide; and a unique capping group, a P3 tertbutyl group on the solvent susceptible side and a trifluoroacetamide occupying the S4 pocket, is designed for better antiviral activity as well as metabolic stability. The optimization from PF-00835231 to PF-07321332 indeed resembles the advanced example on how structural biology, pharmacokinetics and scale-up consideration interact with each other in the process of rational drug development. Tables 1–3 categorize PDB entries of SARS-CoV-1/SARS-CoV-2 main protease structures in complex with peptidomimetic warhead inhibitors, covalent non-peptide inhibitors and noncovalent inhibitors, respectively.
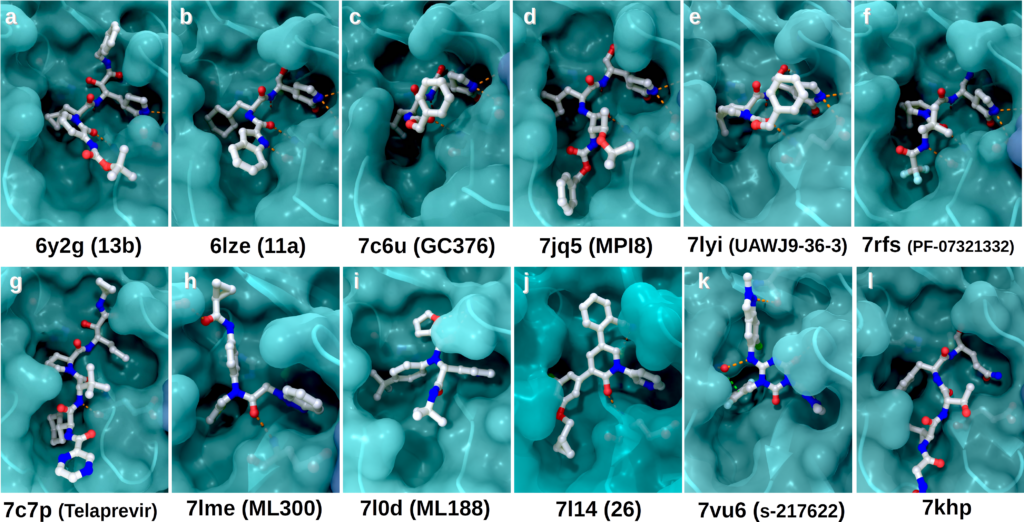
Figure 4. Compounds bound to the active site of SARS-CoV-2; a-b, d-f: peptidomimetic warhead inhibitors, c, g: repurposed drugs, h-k: non-covalent inhibitors, l: physiological substrate. (a) 13b is an α-ketoamide peptidomimetic inhibitor. The P3-P2 amide is replaced by a pyridone ring to increase metabolic stability. (b) 11b is an aldehyde peptidomimetic inhibitor. The indole group at P3 improves metabolic stability and forms an extra hydrogen bond with Glu166. (c) GC376, with a bisulfite warhead, was identified through drug repurposing and is an important lead compound. It has good S1 and S2 interactions but less optimal S3 to S5 capping. (d) MPI8 is a highly potent aldehyde inhibitor. S3 to S5 subsites are effectively capped by the hydrophilic groups at P3 and P4. (e) UAWJ9-36-3 was designed through a hybrid approach. The P1 and P3 groups are adapted from GC376 (c), whereas the P2 and P2/P3 amide are altered to a 6,6-dimethyl-3-azabicyclo[3,0,1]hexane as in boceprivir. (f) PF-07321332 is a nitrile peptidomimetic inhibitor and an approved drug. The P1 and P2 moieties are the same as the ones in UAWJ9-36-3 (e). The trifluoroacetamide effectively caps the S4 pocket. Together with the tert-butyl, the capping group achieves an improved S3 interaction. (g) Telaprevir is an example of α- ketoamide-bearing serine inhibitors identified through drug repurposing. (h, i) ML300 and ML188 are non-covalent inhibitors identified through virtual screening. (j) compound 26 is a highly potent noncovalent inhibitor being optimized from perampanel. (k) s-217622 is a highly potent non-covalent oral drug candidate under clinical trials. The catalytic pole His41 is flipped away from Cys145 as a result of the π–π stacking between the trifluorobenzylic moiety and His41 and the hydrogen bonds mediated by a water molecule. (l) An acyl-enzyme intermediate structure with the C-terminal autoprocessing site bound and Gln306 covalently bound to Cys145. The P5 to P1 residues are shown. The S1, S1, S2, S3 and S4 subsites are highlighted. The arrows indicate the His41–Cys145 catalytic dyad. Molecular surface representations were rendered using Protein Imager [173]. Creator: Coronavirus Structural Task Force - Yunyun Gao, License: cc-by-sa
4. Summary
The overall conformation of the main protease is considered stable; however, the binding site is highly flexible. The important role of the main protease in the viral life cycle and its structural conservation makes it one of the primary targets for antiviral drug development. The structural optimization of peptidomimetic warhead inhibitors as well as high-throughput screens using large compound libraries have generated valuable information for drug development against COVID-19. Crystal structures of the complexes with potent inhibitors have revealed variable binding modes and even mechanistic features, providing crucial insights for drug discovery. However, the inherent flexibility of the binding sites may lead to the emergence of promiscuous inhibitors requiring rigorous further inspection, as do drug candidates interfering with the dimerization interface between the two monomers.
5. Discussion and outlook
During the on-going pandemic, large numbers of sarbecovirus main protease crystal structures have been determined and published, in both apo and intermediate forms, aswell as in complexes with various compounds.While the number of available structures is large, few newstudies focus on revealing the enzymemechanism. It iswell accepted that theN-finger, consisting of only a few key residues, is essential to form the active quaternary structure. However, discussions on the underlying mechanistic details are inconsistent [46,65], and it remains unclear how the maturation of themain protease proceeds (i.e. the dimerization mechanism) due to a lack of atomic resolution information on the key intermediate states. Considering that only the matured dimer shows enzymatic activity, more attention should be given to capture these intermediate states. Such a study could be incredibly beneficial for exploring new drug design strategies. While structural biology can be an excellent tool for drug development, caution should be exercised in interpreting the pharmacology of the binding compounds. Some noncovalent inhibitors with distinct binding modes can be non-specific binders [123]. While a reactive group alone is able to form a complex with the enzyme, the binding affinity would still be significantly lower than the natural substrate in the absence of the peptide chain [158], causing a null inhibitory effect or lack of antiviral effect [62]. It is clear that, in addition to biochemical activity, in vivo drug metabolism and pharmacokinetic profiles such as antiviral activity, absorptive permeability, metabolic stability as well as inhibition reversibility should also be taken into consideration in structure-based SARS-CoV-2 drug design. To date, the continuous endeavour to structure-based drug design has inspired two antiviral drug candidates, the reversible covalent inhibitor PF-07321332 and the noncovalent inhibitor s-217622. For both discoveries, drug metabolism and pharmacokinetic profiles are addressed along with the binding affinity. Current studies of the SARS-CoV-2 main protease of prevalent variants of concern, namely mutants K90R (Alpha, Beta, Gamma), G15S (Lambda) and P132H (Omicron), suggest that mutations lead to almost identical backbone and active site conformations [150]. The in vivo antiviral activity of PF-07321332 remains the same for these different variants [134,150,159]. Other prevalent mutations, T21I (Beta), L89F (Beta), L205V (Zeta) also remain susceptible to PF-07321332 in biochemical assays [69]. Unlike the spike protein, where mutations lead to resistance, this treatment is therefore unaffected by currently-observed prevalent mutations, making continued targeting of the main protease a core antiviral therapy against COVID-19. Should resistance arise, led by the use of antiviral monotherapies [160], we may need to explore alternative approaches such as targeting the dimerization interface or directly interfering with the dimerization process. However, very limited results have been reported on dimer-interfering inhibitors, and only a few compounds binding to the dimerization interface were shown by crystallographic fragment screening campaigns [4,5]. Dimerization inhibitors targeting many viral enzymes, including HIV protease, have been reported [161–163], so it is nonetheless likely that inhibitors of main protease inspired by such design strategies will eventually become available. So many models and crystallographic data sets of the same protein, solved in the same relatively short time period, also provide an unique opportunity for computational method developers. The crystallographic data sets can serve as a consistent resource for developing new or improving current crystallography pipelines and methodologies. For this, the dedicated databases aiming to validate and re-refine the deposited coronavirus protein models are of great value, such as covid-19.bioreproducibility.org [164] and the Coronavirus Structural Task Force [165]. Moreover, the structural stability and robust crystallizability of the main protease suggests it can be used as a model system for experimental crystallographic method development as well. Potential use cases include testing protocols of high-throughput drug screening by serial femtosecond X-ray crystallography [166], accessing new screening technologies [167–169] or new experimental compound optimization strategies [170], evaluating the performance of a new fluorescent probes/assays [171,172] and validating the sensitivity of computational inhibitor design pipelines [111].
This blog post was published in Crystallography Reviews, please cite: https://doi.org/10.1080/0889311X.2023.2222275
Acknowledgements
We thank RosemaryWilson for proofreading. All figures are courtesy of the Coronavirus Structural Task Force (insidecorona.net) which retains copyright for both the text and the figures.
References
[1] Anand K, Ziebuhr J, Wadhwani P, et al. Coronavirus main proteinase (3CLpro) structur basis for design of anti-SARS drugs. Science. 2003;300:1763–1767.
[2] Fehr AR, Perlman S. Coronaviruses: an overview of their replication and pathogenesis. Methods Mol Biol. 2015;1282:1–23.
[3] Ziebuhr J, Snijder EJ, Gorbalenya AE. Virus-encoded proteinases and proteolytic processing in the Nidovirales. J Gen Virol. 2000;81:853–879.
[4] Douangamath A, Fearon D, Gehrtz P, et al. Crystallographic and electrophilic fragment screening of the SARS-CoV-2 main protease. Nat Commun. 2020;11:5047.
[5] Günther S, Reinke PYA, Fernández-García Y, et al. X-ray screening identifies active site and allosteric inhibitors of SARS-CoV-2 main protease. Science. 2021;372:642–646.
[6] Matthews DA, Smith WW, Ferre RA, et al. Structure of human rhinovirus 3C protease reveals a trypsin-like polypeptide fold, RNA-binding site, and means for cleaving precursor polyprotein. Cell. 1994;77:761–771.
[7] Mosimann SC, Cherney MM, Sia S, et al. Refined X-ray crystallographic structure of the poliovirus 3C gene product. J Mol Biol. 1997;273:1032–1047.
[8] Hegyi A, Ziebuhr J.Conservation of substrate specificities among coronavirus main proteases.J Gen Virol. 2002;83:595–599.
[9] Hegyi A, Friebe A, Gorbalenya AE, et al. Mutational analysis of the active centre of coronavirus 3C-like proteases. J Gen Virol. 2002;83:581–593.
[10] Ziebuhr J, Heusipp G, Siddell SG. Biosynthesis,: purification, and characterization of the human coronavirus 229E 3C-like proteinase. J Virol. 1997;71:3992–3997.
[11] Anand K, Palm GJ, Mesters JR, et al. Structure of coronavirus main proteinase reveals combination of a chymotrypsin fold with an extra alpha-helical domain. EMBO J. 2002;21:3213–3224.
[12] Drosten C, Günther S, Preiser W, et al. Identification of a novel coronavirus in patients with severe acute respiratory syndrome. N Engl J Med. 2003;348:1967–1976.
[13] Ksiazek TG, ErdmanD, Goldsmith CS, et al. A novel coronavirus associated with severe acute respiratory syndrome. N Engl J Med. 2003;348:1953–1966.
[14] Ziebuhr J. Molecular biology of severe acute respiratory syndrome coronavirus. Curr Opin Microbiol. 2004;7:412–419.
[15] Yang H, Yang M, Ding Y, et al. The crystal structures of severe acute respiratory syndrome virus main protease and its complex with an inhibitor. Proc Natl Acad Sci USA. 2003;100:13190–13195.
[16] Jin Z, Du X, Xu Y, et al. Structure of Mpro from SARS-CoV-2 and discovery of its inhibitors. Nature. 2020;582:289–293.
[17] Qin E, ZhuQ,Yu M, et al.Acomplete sequence and comparative analysis of a SARS-associated virus (Isolate BJ01). Chin Sci Bull. 2003;48:941–948.
[18] Hsu M-F, Kuo C-J, Chang K-T, et al.Mechanism of the maturation process of SARS-CoV 3CL protease. J Biol Chem. 2005;280:31257–31266.
[19] Ghosh AK, Xi K, Ratia K, et al. Design and synthesis of peptidomimetic severe acute respiratory syndrome chymotrypsin-like protease inhibitors. J Med Chem. 2005;48:6767–6771.
[20] Pyrc K, Berkhout B, van der Hoek L. The novel human coronaviruses NL63 and HKU1. J Virol. 2007;81:3051–3057.
[21] Zaki AM, van Boheemen S, Bestebroer TM, et al. Isolation of a novel coronavirus fromaman with pneumonia in Saudi Arabia. N Engl J Med. 2012;367:1814–1820.
[22] Shi J, Sivaraman J, Song J. Mechanism for controlling the dimer-monomer switch and coupling dimerization to catalysis of the severe acute respiratory syndrome coronavirus 3C-like protease. J Virol. 2008;82:4620–4629.
[23] Tan J, Verschueren KHG, Anand K, et al. pH-dependent conformational flexibility of the SARS-CoV main proteinase (M(pro)) dimer: molecular dynamics simulations and multiple X-ray structure analyses. J Mol Biol. 2005;354:25–40.
[24] Xue X, Yu H, Yang H, et al. Structures of two coronavirus main proteases: implications for substrate binding and antiviral drug design. J Virol. 2008;82:2515–2527.
[25] Hu T, Zhang Y, Li L, et al. Two adjacent mutations on the dimer interface of SARS coronavirus 3C-like protease cause different conformational changes in crystal structure. Virology.2009;388:324–334.
[26] Barrila J, Gabelli SB, Bacha U, et al. Mutation of Asn28 disrupts the dimerization and enzymatic activity of SARS 3CL(pro). Biochemistry. 2010;49:4308–4317.
[27] Muramatsu T, Takemoto C, Kim Y-T, et al. SARS-CoV 3CL protease cleaves its Cterminal autoprocessing site by novel subsite cooperativity. Proc Natl Acad Sci USA.2016;113:12997–13002.
[28] Yang H, XieW, Xue X, et al. Design of wide-spectrum inhibitors targeting coronavirus main proteases. PLoS Biol. 2005;3:e324.
[29] Lee T-W, Cherney MM, Huitema C, et al. Crystal structures of the main peptidase from the SARS coronavirus inhibited by a substrate-like aza-peptide epoxide. J Mol Biol.2005;353:1137–1151.
[30] Lu I-L, Mahindroo N, Liang P-H, et al. Structure-based drug design and structural biology study of novel nonpeptide inhibitors of severe acute respiratory syndrome coronavirus main protease. J Med Chem. 2006;49:5154–5161.
[31] Verschueren KHG, Pumpor K, Anemüller S, et al. A structural view of the inactivation of the SARS coronavirus main proteinase by benzotriazole esters. Chem Biol. 2008;15:597–606.
[32] Akaji K, Konno H, Mitsui H, et al. Structure-based design, synthesis, and evaluation of peptide-mimetic SARS 3CL protease inhibitors. J Med Chem. 2011;54:7962–7973.
[33] Zhu L, George S, Schmidt MF, et al. Peptide aldehyde inhibitors challenge the substrate specificity of the SARS-coronavirus main protease. Antiviral Res. 2011;92:204–212.
[34] Jacobs J,Grum-TokarsV, ZhouY, et al.Discovery, synthesis, and structure-based optimization of a series of N-(tert-butyl)-2-(N-arylamido)-2-(pyridin-3-yl) acetamides (ML188) as potent noncovalent small molecule inhibitors of the severe acute respiratory syndrome coronavirus (SARS-CoV) 3CL protease. J Med Chem. 2013;56:534–546.
[35] Chuck C-P, Chen C, Ke Z, et al. Design, synthesis and crystallographic analysis of nitrilebased broad-spectrum peptidomimetic inhibitors for coronavirus 3C-like proteases. Eur J Med Chem. 2013;59:1–6.
[36] Turlington M, Chun A, Tomar S, et al. Discovery of N-(benzo[1,2,3]triazol-1-yl)-N-(benzyl)acetamido)phenyl) carboxamides as severe acute respiratory syndrome coronavirus (SARS-CoV) 3CLpro inhibitors: identification of ML300 and noncovalent nanomolar inhibitors with an induced-fit binding. Bioorg Med Chem Lett. 2013;23:6172–6177.
[37] Shimamoto Y, Hattori Y, Kobayashi K, et al. Fused-ring structure of decahydroisoquinolin as a novel scaffold for SARS 3CL protease inhibitors. Bioorg Med Chem. 2015;23:876–890.
[38] Wu F, Zhao S, Yu B, et al. A new coronavirus associated with human respiratory disease in China. Nature. 2020;579:265–269.
[39] Roe MK, Junod NA, Young AR, et al. Targeting novel structural and functional features of coronavirus protease nsp5 (3CLpro, Mpro) in the age of COVID-19. J Gen Virol. 2021;102:001558.
[40] Luttens A, Gullberg H, Abdurakhmanov E, et al. Ultralarge virtual screening identifies SARSCoV-2 main protease inhibitors with broad-spectrum activity against coronaviruses. J Am Chem Soc. 2022;144:2905–2920.
[41] Antonopoulou I, Sapountzaki E, Rova U, et al. Inhibition of themain protease of SARS-CoV-2 (Mpro) by repurposing/designing drug-like substances and utilizing nature’s toolbox of bioactive compounds. Comput Struct Biotechnol J. 2022;20:1306–1344.
[42] Jaskolski M, Dauter Z, Shabalin IG, et al. Crystallographic models of SARS-CoV-2 3CLpro: in-depth assessment of structure quality and validation. IUCrJ. 2021;8:238–256.
[43] Tekpinar M, Yildirim A. Impact of dimerization and N3 binding on molecular dynamics of SARS-CoV and SARS-CoV-2 main proteases. J Biomol Struct Dyn. 2022;40:6243–6254.
[44] Wang H,He S, DengW, et al. Comprehensive insights into the catalytic mechanism of Middle East respiratory syndrome 3C-like protease and severe acute respiratory syndrome 3C-like
protease. ACS Catal. 2020;10:5871–5890.
[45] Komatsu TS, Okimoto N, Koyama YM, et al. Drug binding dynamics of the dimeric SARSCoV-2main protease, determined bymolecular dynamics simulation. Sci Rep. 2020;10:16986.
[46] Xiong M, Su H, Zhao W, et al. What coronavirus 3C-like protease tells us: from structure, substrate selectivity, to inhibitor design. Med Res Rev. 2021;41:1965–1998.
[47] Huang C, Wei P, Fan K, et al. 3C-like proteinase from SARS coronavirus catalyzes substrate hydrolysis by a general base mechanism. Biochemistry. 2004;43:4568–4574.
[48] Paasche A, Zipper A, Schäfer S, et al. Evidence for substrate binding-induced zwitterion formation in the catalytic Cys-His dyad of the SARS-CoV main protease. Biochemistry.2014;53:5930–5946.
[49] Kneller DW, Phillips G, Weiss KL, et al. Unusual zwitterionic catalytic site of SARS-CoV-2 main protease revealed by neutron crystallography. J Biol Chem. 2020;295:17365–17373.
[50] Kneller DW, Phillips G, O’Neill HM, et al. Room-temperature X-ray crystallography reveals the oxidation and reactivity of cysteine residues in SARS-CoV-2 3CL Mpro: insights into enzyme mechanism and drug design. IUCrJ. 2020;7:1028–1035.
[51] Lee J, Worrall LJ, Vuckovic M, et al. Crystallographic structure of wild-type SARS-CoV-2 main protease acyl-enzyme intermediate with physiological C-terminal autoprocessing site. Nat Commun. 2020;11:5877.
[52] Kneller DW, Phillips G, O’Neill HM, et al. Structural plasticity of SARS-CoV-2 3CL Mpro active site cavity revealed by room temperature X-ray crystallography. Nat Commun. 2020;11:3202.
[53] Ménard R, Storer AC.Oxyanion hole interactions in serine and cysteine proteases. Biol Chem Hoppe-Seyler. 1992;373:393–400.
[54] Chen S, Hu T, Zhang J, et al. Mutation of Gly-11 on the dimer interface results in the complete crystallographic dimer dissociation of severe acute respiratory syndrome coronavirus 3C-like protease: crystal structure with molecular dynamics simulations. J Biol Chem. 2008;283:554–564.
[55] DaiW, Zhang B, JiangX-M, et al. Structure-based design of antiviral drug candidates targeting the SARS-CoV-2 main protease. Science. 2020;368:1331–1335.
[56] Hattori S-I, Higashi-Kuwata N, HayashiH, et al. A small molecule compound with an indole moiety inhibits themain protease of SARS-CoV-2 and blocks virus replication. Nat Commun.2021;12:668.
[57] Yang KS, Ma XR, Ma Y, et al. A quick route to multiple highly potent SARS-CoV-2 main protease inhibitors. ChemMedChem. 2021;16:942–948.
[58] MacDonald EA, Frey G, NamchukMN, et al. Recognition of divergent viral substrates by the SARS-CoV-2 main protease. ACS Infect Dis. 2021;7:2591–2595.
[59] Hoffman RL, Kania RS, Brothers MA, et al. Discovery of ketone-based covalent inhibitors
of coronavirus 3CL proteases for the potential therapeutic treatment of COVID-19. J Med Chem. 2020;63:12725–12747.
[60] Ghosh AK, Xi K, Grum-Tokars V, et al. Structure-based design, synthesis, and biological evaluation of peptidomimetic SARS-CoV 3CLpro inhibitors. Bioorg Med Chem Lett. 2007;17:5876–5880.
[61] Zhang C-H, Stone EA, Deshmukh M, et al. Potent noncovalent inhibitors of the main protease of SARS-CoV-2 frommolecular sculpting of the drug perampanel guided by free energy
perturbation calculations. ACS Cent Sci. 2021;7:467–475.
[62] Zhang L, Lin D, Kusov Y, et al. α-Ketoamides as broad-spectrum inhibitors of coronavirus
and enterovirus replication: structure-based design, synthesis, and activity assessment. JMed Chem. 2020;63:4562–4578.
[63] HsuW-C,Chang H-C, Chou C-Y, et al. Critical assessment of important regions in the subunit association and catalytic action of the severe acute respiratory syndrome coronavirus main protease. J Biol Chem. 2005;280:22741–22748.
[64] Chou C-Y, Chang H-C, HsuW-C, et al. Quaternary structure of the severe acute respiratory syndrome (SARS) coronavirus main protease. Biochemistry. 2004;43:14958–14970.
[65] Zhong N, Zhang S, Zou P, et al. Without itsN-finger, the main protease of severe acute respiratory syndrome coronavirus can forma novel dimer through its C-terminal domain. J Virol.2008;82:4227–4234.
[66] Zhong N, Zhang S, Xue F, et al. C-terminal domain of SARS-CoV main protease can form a 3D domain-swapped dimer. Protein Sci. 2009;18:839–844.
[67] Chen S, Zhang J, Hu T, et al. Residues on the dimer interface of SARS coronavirus 3C-like protease: dimer stability characterization and enzyme catalytic activity analysis. J Biochem. 2008;143:525–536.
[68] Shan Y-F, Li S-F, Xu G-J. A novel auto-cleavage assay for studying mutational effects on the active site of severe acute respiratory syndrome coronavirus 3C-like protease. Biochem Biophys Res Commun. 2004;324:579–583.
[69] Ullrich S, Ekanayake KB, Otting G, et al. Main protease mutants of SARS-CoV-2 variants remain susceptible to nirmatrelvir. Bioorg Med Chem Lett. 2022;62:128629.
[70] Lin P-Y, Chou C-Y, Chang H-C, et al. Correlation between dissociation and catalysis of SARSCoV main protease. Arch Biochem Biophys. 2008;472:34–42.
[71] Noske GD, Nakamura AM, Gawriljuk VO, et al. A crystallographic snapshot of SARS-CoV-2 main protease maturation process. J Mol Biol. 2021;433:167118.
[72] Sun Z, Wang L, Li X, et al. An extended conformation of SARS-CoV-2main protease reveals allosteric targets. Proc Natl Acad Sci USA. 2022;119:e2120913119.
[73] Ebrahim A, Riley BT, Kumaran D, et al. The temperature-dependent conformational ensemble of SARS-CoV-2 main protease (M pro). BioRxiv. 2021.
[74] Pearce NM, Gros P. A method for intuitively extracting macromolecular dynamics from structural disorder. Nat Commun. 2021;12:5493.
[75] Ghosh AK, Osswald HL, Prato G. Recent progress in the development of HIV-1 protease inhibitors for the treatment of HIV/AIDS. J Med Chem. 2016;59:5172–5208.
[76] de LeuwP, StephanC. Protease inhibitors for the treatment ofhepatitisCvirus infection. GMS Infect Dis. 2017;5:Doc08.
[77] Zephyr J, Kurt Yilmaz N, Schiffer CA. Viral proteases: structure, mechanism and inhibition. Enzymes. 2021;50:301–333.
[78] Lee C-C, Kuo C-J, Ko T-P, et al. Structural basis of inhibition specificities of 3C and 3C-like proteases by zinc-coordinating and peptidomimetic compounds. J Biol Chem.
2009;284:7646–7655.
[79] Lee T-W, Cherney MM, Liu J, et al. Crystal structures reveal an induced-fit binding of a substrate-like Aza-peptide epoxide to SARS coronavirus main peptidase. J Mol Biol. 2007;366:916–932.
[80] Yang S, Chen S-J, Hsu M-F, et al. Synthesis, crystal structure, structure-activity relationships, and antiviral activity of a potent SARS coronavirus 3CL protease inhibitor. J Med Chem. 2006;49:4971–4980.
[81] Bai B, Belovodskiy A, Hena M, et al. Peptidomimetic α-acyloxymethylketone warheads with six-membered lactam P1 glutamine mimic: SARS-CoV-2 3CL protease inhibition, coronavirus antiviral activity, and in vitro biological stability. J Med Chem. 2022;65:2905–2925.
[82] Yin J, NiuC, CherneyMM, et al. Amechanistic viewof enzyme inhibitionandpeptide hydrolysis in the active site of the SARS-CoV3C-like peptidase. J Mol Biol. 2007;371:1060–1074.
[83] Zhang L, Lin D, Sun X, et al. Crystal structure of SARS-CoV-2 main protease provides a basis for design of improved α-ketoamide inhibitors. Science. 2020;368:409–412.
[84] Rathnayake AD, Zheng J, Kim Y, et al. 3C-like protease inhibitors block coronavirus replication in vitro and improve survival in MERS-CoV-infected mice. Sci Transl Med. 2020;12:eabc5332.
[85] Dampalla CS, Kim Y, Bickmeier N, et al. Structure-guided design of conformationally constrained cyclohexane inhibitors of severe acute respiratory syndrome coronavirus-2 3CL protease. J Med Chem. 2021;64:10047–10058.
[86] Dampalla CS, Rathnayake AD, Perera KD, et al. Structure-guided design of potent inhibitors of SARS-CoV-2 3CL protease: structural, biochemical, and cell-based studies. J Med Chem. 2021;64:17846–17865.
[87] Vuong W, Khan MB, Fischer C, et al. Feline coronavirus drug inhibits the main protease of SARS-CoV-2 and blocks virus replication. Nat Commun. 2020;11:4282.
[88] Sacco MD, Ma C, Lagarias P, et al. Structure and inhibition of the SARS-CoV-2 main protease reveal strategy for developing dual inhibitors against Mpro and cathepsin L. Sci Adv. 2020;6:eabe0751.
[89] Fu L, Ye F, Feng Y, et al. Both Boceprevir and GC376 efficaciously inhibit SARS-CoV-2 by targeting its main protease. Nat Commun. 2020;11:4417.
[90] Ma C, Sacco MD, Hurst B, et al. Boceprevir, GC-376, and calpain inhibitors II, XII inhibit SARS-CoV-2 viral replication by targeting the viralmain protease. Cell Res. 2020;30:678–692.
[91] Arutyunova E, Khan MB, Fischer C, et al. N-Terminal finger stabilizes the S1 pocket for the reversible feline drug GC376 in the SARS-CoV-2 Mpro dimer. J Mol Biol. 2021;433:167003.
[92] Shi Y, Shuai L,Wen Z, et al. The preclinical inhibitor GS441524 in combination with GC376 efficaciously inhibited the proliferation of SARS-CoV-2 in themouse respiratory tract. Emerg Microbes Infect. 2021;10:481–492.
[93] VuongW, FischerC, KhanMB, et al. Improved SARS-CoV-2Mpro inhibitors based on feline antiviral drug GC376: structural enhancements, increased solubility, and micellar studies. Eur J Med Chem. 2021;222:113584.
[94] Liu H, Iketani S, Zask A, et al. Development of optimized drug-like smallmolecule inhibitors of the SARS-CoV-2 3CL protease for treatment of COVID-19. Nat Commun. 2022;13:1891.
[95] Bacha U, Barrila J, Gabelli SB, et al. Development of broad-spectrum halomethyl ketone inhibitors against coronavirus main protease 3CL(pro). Chem Biol Drug Des. 2008;72:34–49.
[96] Ullrich S, Sasi VM, MahawaththaMC, et al. Challenges of short substrate analogues as SARSCoV-2 main protease inhibitors. BioorgMed Chem Lett. 2021: 128333.
[97] Amporndanai K, Meng X, Shang W, et al. Inhibition mechanism of SARS-CoV-2 main protease by ebselen and its derivatives. Nat Commun. 2021;12:3061.
[98] Su H, Yao S, Zhao W, et al. Identification of pyrogallol as a warhead in design of covalent inhibitors for the SARS-CoV-2 3CL protease. Nat Commun. 2021;12:3623.
[99] Kitamura N, Sacco MD, Ma C, et al. Expedited approach toward the rational design of noncovalent SARS-CoV-2 main protease inhibitors. J Med Chem. 2022;65:2848–2865.
[100] Mslati H, Gentile F, Perez C, et al. Comprehensive consensus analysis of SARS-CoV-2 drug repurposing campaigns. J Chem Inf Model. 2021;61:3771–3788.
[101] Lockbaum GJ, Reyes AC, Lee JM, et al. Crystal structure of SARS-CoV-2 main protease in complex with the non-covalent inhibitor ML188. Viruses. 2021;13.
[102] Deshmukh MG, Ippolito JA, Zhang C-H, et al. Structure-guided design of a perampanelderived pharmacophore targeting the SARS-CoV-2 main protease. Structure. 2021;29:
823–833.e5.
[103] Clyde A, Galanie S, Kneller DW, et al. High-throughput virtual screening and validation of a SARS-CoV-2 main protease noncovalent inhibitor. J Chem Inf Model. 2022;62:116–128.
[104] RedheadMA, Owen CD, Brewitz L, et al. Bispecific repurposed medicines targeting the viral and immunological arms of COVID-19. Sci Rep. 2021;11:13208.
[105] Iketani S, Forouhar F, Liu H, et al. Lead compounds for the development of SARS-CoV-2 3CL protease inhibitors. Nat Commun. 2021;12:2016.
[106] Gimeno A, Mestres-Truyol J, Ojeda-Montes MJ, et al. Prediction of novel inhibitors of the main protease (M-pro) of SARS-CoV-2 through consensus docking and drug reposition. Int J Mol Sci. 2020;21:3793.
[107] Han SH, Goins CM, Arya T, et al. Structure-based optimization of ML300-derived, noncovalent inhibitors targeting the severe acute respiratory syndrome coronavirus 3CL protease (SARS-CoV-2 3CLpro). J Med Chem. 2022;65:2880–2904.
[108] Llanos MA, Gantner ME, Rodriguez S, et al. Strengths and weaknesses of docking simulations in the SARS-CoV-2 era: the main protease (Mpro) case study. J Chem Inf Model. 2021;61:3758–3770.
[109] Tanaka S, Tokutomi S, Hatada R, et al. Dynamic cooperativity of ligand-residue interactions evaluated with the fragment molecular orbital method. J Phys Chem B. 2021;125:6501–6512.
[110] Stille JK, Tjutrins J, Wang G, et al. Design, synthesis and in vitro evaluation of novel SARSCoV-2 3CLpro covalent inhibitors. Eur J Med Chem. 2022;229:114046.
[111] Zaidman D, Gehrtz P, Filep M, et al. An automatic pipeline for the design of irreversible derivatives identifies a potent SARS-CoV-2 Mpro inhibitor. Cell Chem Biol. 2021;28:1795–1806.e5.
[112] Unoh Y, Uehara S, Nakahara K, et al. Discovery of S-217622, a noncovalent oral SARSCoV-2 3CL protease inhibitor clinical candidate for treating COVID-19. J Med Chem. 2022;65:6499–6512.
[113] Shionogi Co., Ltd. New Data for Shionogi’s COVID-19 Once-Daily Oral Antiviral S-217622 Show Rapid Virus Clearance. News. Shionogi Co., Ltd. [Internet]. [cited 2022 May 3].
Available from: https://www.shionogi.com/global/en/news/2022/04/20220424.html.
[114] Su H-X, Yao S, Zhao W-F, et al. Anti-SARS-CoV-2 activities in vitro of Shuanghuanglian preparations and bioactive ingredients. Acta Pharmacol Sin. 2020;41:1167–1177.
[115] Jin Z, Zhao Y, Sun Y, et al. Structural basis for the inhibition of SARS-CoV-2 main protease by antineoplastic drug carmofur. Nat Struct Mol Biol. 2020;27:529–532.
[116] Li J, Zhou X, Zhang Y, et al. Crystal structure of SARS-CoV-2 main protease in complex with the natural product inhibitor shikonin illuminates a unique binding mode. Sci Bull (Beijing).2021;66:661–663.
[117] Kneller DW, Galanie S, Phillips G, et al. Malleability of the SARS-CoV-2 3CLMpro active-site cavity facilitates binding of clinical antivirals. Structure. 2020;28:1313–1320.e3.
[118] Bai Y, Ye F, Feng Y, et al. Structural basis for the inhibition of the SARS-CoV-2 main protease by the anti-HCV drug narlaprevir. Signal Transduct Target Ther. 2021;6:51.
[119] Qiao J, Li Y-S, Zeng R, et al. SARS-CoV-2 Mpro inhibitors with antiviral activity in a transgenic mouse model. Science. 2021;371:1374–1378.
[120] Oerlemans R, Ruiz-Moreno AJ, Cong Y, et al. Repurposing the HCV NS3-4A protease drug boceprevir as COVID-19 therapeutics. RSC Med Chem. 2020;12:370–379.
[121] Drayman N, DeMarco JK, Jones KA, et al. Masitinib is a broad coronavirus 3CL inhibitor that blocks replication of SARS-CoV-2. Science. 2021;373:931–936.
[122] Dementiev A, Joachimiak A, Nguyen H, et al. Molecular mechanism of inhibition of acid ceramidase by carmofur. J Med Chem. 2019;62:987–992.
[123] Ma C, Hu Y, Townsend JA, et al. Ebselen, disulfiram, carmofur, PX-12, tideglusib, and shikonin Are nonspecific promiscuous SARS-CoV-2 main protease inhibitors. ACS Pharmacol Transl Sci. 2020;3:1265–1277.
[124] Baker JD, Uhrich RL, Kraemer GC, et al. A drug repurposing screen identifies hepatitis C antivirals as inhibitors of the SARS-CoV2 main protease. PLoS ONE. 2021;16:e0245962.
[125] Xia Z, Sacco M, Hu Y, et al. Rational design of hybrid SARS-CoV-2 main protease inhibitors guided by the superimposed cocrystal structures with the peptidomimetic inhibitors GC-376, telaprevir, and boceprevir. ACS Pharmacol Transl Sci. 2021;4:1408–1421.
[126] Owen DR, Allerton CMN, Anderson AS, et al. An oral SARS-CoV-2 Mpro inhibitor clinical candidate for the treatment of COVID-19. Science. 2021;374:1586–1593.
[127] Abdelnabi R, Foo CS, Jochmans D, et al. The oral protease inhibitor (PF-07321332) protects Syrian hamsters against infection with SARS-CoV-2 variants of concern. Nat Commun.2022;13:719.
[128] Li J, Lin C, Zhou X, et al. Structural basis of the main proteases of coronavirus bound to drug candidate PF-07321332. J Virol. 2022;96:e0201321.
[129] Goetz DH, Choe Y, Hansell E, et al. Substrate specificity profiling and identification of a new class of inhibitor for the major protease of the SARS coronavirus. Biochemistry.2007;46:8744–8752.
[130] Konno S,Kobayashi K, Senda M, et al. 3CL protease inhibitorswith an electrophilic arylketone moiety as anti-SARS-CoV-2 agents. J Med Chem. 2022;65:2926–2939.
[131] Li J, Lin C, Zhou X, et al. Structural basis of main proteases of coronavirus bound to drug candidate PF-07304814. J Mol Biol. 2022;434:167706.
[132] Ma Y, Yang KS, Geng ZZ, et al. A multi-pronged evaluation of aldehyde-based tripeptidyl main protease inhibitors as SARS-CoV-2 antivirals. Eur J Med Chem. 2022;240:114570.
[133] Bai B, Arutyunova E, Khan MB, et al. Peptidomimetic nitrile warheads as SARS-CoV-2 3CL protease inhibitors. RSC Med Chem. 2021;12:1722–1730.
[134] Sacco MD, Hu Y, Gongora MV, et al. The P132H mutation in the main protease of Omicron SARS-CoV-2 decreases thermal stability without compromising catalysis or small-molecule drug inhibition. Cell Res. 2022;32:498–500.
[135] Dampalla CS, Rathnayake AD, Galasiti Kankanamalage AC, et al. Structure-guided design of potent spirocyclic inhibitors of severe acute respiratory syndrome coronavirus-2 3C-like protease. J Med Chem. 2022;65:7818–7832.
[136] Lee C-C, Kuo C-J,Hsu M-F, et al. Structural basis ofmercury- and zinc-conjugated complexes as SARS-CoV 3C-like protease inhibitors. FEBS Lett. 2007;581:5454–5458.
[137] Pillaiyar T, Flury P, Krüger N, et al. Small-molecule thioesters as SARS-CoV-2 main protease inhibitors: enzyme inhibition, structure-activity relationships, antiviral activity, and X-ray structure determination. J Med Chem. 2022;65:9376–9395.
[138] Fu L, Shao S, Feng Y, et al. Mechanism of microbial metabolite leupeptin in the treatment of COVID-19 by traditional Chinese medicine herbs. MBio. 2021;12:e0222021.
[139] Andi B,KumaranD,KreitlerDF, et al. HepatitisCvirus NS3/4A inhibitors and other drug-like compounds as covalent binders of SARS-CoV-2 main protease. Sci Rep. 2022;12:12197.
[140] Kneller DW, Phillips G, Weiss KL, et al. Direct observation of protonation state modulation in SARS-CoV-2 main protease upon inhibitor binding with neutron crystallography. J Med Chem. 2021;64:4991–5000.
[141] Lockbaum GJ, Henes M, Lee JM, et al. Pan-3C protease inhibitor rupintrivir binds SARSCoV-2 main protease in a unique bindingmode. Biochemistry. 2021;60:2925–2931.
[142] Kuzikov M, Costanzi E, Reinshagen J, et al. Identification of inhibitors of SARS-CoV-2 3CLPro enzymatic activity using a small molecule in vitro repurposing screen. ACS Pharmacol Transl Sci. 2021;4:1096–1110.
[143] Costanzi E, Kuzikov M, Esposito F, et al. Structural and biochemical analysis of the dual inhibition of MG-132 against SARS-CoV-2 main protease (Mpro/3CLpro) and human cathepsin-L. Int J Mol Sci. 2021;22:11779.
[144] Ghosh AK, Raghavaiah J, Shahabi D, et al. Indole chloropyridinyl ester-derived SARS-CoV-2 3CLpro inhibitors: enzyme inhibition, antiviral efficacy, structure-activity relationship, and X-ray structural studies. J Med Chem. 2021;64:14702–14714.
[145] Malla TR, Brewitz L,Muntean D-G, et al. Penicillin derivatives inhibit the SARS-CoV-2 main protease by reaction with its nucleophilic cysteine. J Med Chem. 2022;65:7682–7696.
[146] Ma C, Xia Z, SaccoMD, et al. Discovery of di- and trihaloacetamides as covalent SARS-CoV-2 main protease inhibitors with high target specificity. JAmChem Soc. 2021;143:20697–20709.
[147] Alugubelli YR, Geng ZZ, Yang KS, et al. A systematic exploration of boceprevir-based main protease inhibitors as SARS-CoV-2 antivirals. Eur J Med Chem. 2022;240:114596.
[148] Zhao Y, Fang C, Zhang Q, et al. Crystal structure of SARS-CoV-2 main protease in complex with protease inhibitor PF-07321332. Protein Cell. 2022;13:689–693.
[149] Kneller D, Li H, Phillips G, et al. Covalent narlaprevir- and boceprevir-derived hybrid inhibitors of SARS-CoV-2 main protease: room-temperature X-ray and neutron crystallography, binding thermodynamics, and antiviral activity. [Preprint]. Res Sq. 2022.
[150] Greasley SE,Noell S, PlotnikovaO, et al. Structural basis for the in vitro efficacy of nirmatrelvir against SARS-CoV-2 variants. J Biol Chem. 2022: 101972.
[151] XiongM, Nie T, Shao Q, et al. In silico screening-based discovery of novel covalent inhibitors of the SARS-CoV-2 3CL protease. Eur J Med Chem. 2022;231:114130.
[152] Cantrelle F-X, Boll E, Brier L, et al. NMR spectroscopy of the main protease of SARS-CoV-2 and fragment-based screening identify three protein hotspots and an antiviral fragment.Angew Chem Int Ed. 2021;60:25428–25435.
[153] Kneller DW, Li H, Galanie S, et al. Structural, electronic, and electrostatic determinants for inhibitor binding to subsites S1 and S2 in SARS-CoV-2 main protease. J Med Chem.2021;64:17366–17383.
[154] Zhong B, Peng W, Du S, et al. Oridonin inhibits sars-cov-2 by targeting its 3c-like protease.Small Sci. 2022;2:2100124.
[155] Rossetti GG, Ossorio MA, Rempel S, et al. Non-covalent SARS-CoV-2 Mpro inhibitors developed from in silico screen hits. Sci Rep. 2022;12:2505.
[156] Zhang Y, Gao H, Hu X, et al. Structure-based discovery and structural basis of a novel broad-spectrum natural product against the main protease of coronavirus. J Virol. 2022;96:e0125321.
[157] Yang KS, Alex Kuo S-T, Blankenship LR, et al. Repurposing halicin as a potent covalent inhibitor for the SARS-CoV-2 main protease. Curr Res Chem Biol. 2022;2:100025.
[158] Shaqra AM, Zvornicanin SN, Huang QYJ, et al. Defining the substrate envelope of SARSCoV-2 main protease to predict and avoid drug resistance. Nat Commun. 2022;13:3556.
[159] Vangeel L, Chiu W, De Jonghe S, et al. Remdesivir, Molnupiravir and Nirmatrelvir remain active against SARS-CoV-2 omicron and other variants of concern. Antiviral Res.2022;198:105252.
[160] Sidebottom DB, Smith DD, Gill D. Safety and efficacy of antivirals against SARS-CoV-2. Br Med J. 2021;375:n2611.
[161] Ye C, Bian P, Zhang J, et al. Structure-based discovery of antiviral inhibitors targeting the E dimer interface of Japanese encephalitis virus. Biochem Biophys Res Commun.2019;515:366–371.
[162] Pietrucci F, Vargiu AV, Kranjc A. HIV-1 Protease dimerization dynamics reveals a transient druggable binding pocket at the interface. Sci Rep. 2015;5:18555.
[163] Bannwarth L, Kessler A, Pèthe S, et al. Molecular tongs containing amino acid mimetic fragments:new inhibitors of wild-type and mutated HIV-1 protease dimerization. J Med Chem.2006;49:4657–4664.
[164] Brzezinski D, Kowiel M, Cooper DR, et al. Covid-19.bioreproducibility.org: A web resource for SARS-CoV-2-related structural models. Protein Sci. 2021;30:115–124.
[165] Croll TI, Diederichs K, Fischer F, et al. Making the invisible enemy visible. Nat Struct Mol Biol. 2021;28:404–408.
[166] GuvenO,Gul M,Ayan E, et al. Case study of high-throughput drug screening and remote data collection for SARS-CoV-2main protease by using serial femtosecond X-ray crystallography.Crystals. 2021;11:1579.
[167] Chamakuri S, Lu S, Ucisik MN, et al. DNA-encoded chemistry technology yields expedient access to SARS-CoV-2 Mpro inhibitors. Proc Natl Acad Sci USA. 2021;118:e2111172118.
[168] Gildea RJ, Beilsten-Edmands J, Axford D, et al. Xia2.multiplex: a multi-crystal data-analysis pipeline. Acta Crystallogr D Struct Biol. 2022;78:752–769.
[169] Narayanan A, Narwal M, Majowicz SA, et al. Identification of SARS-CoV-2 inhibitors targeting Mpro and PLpro using in-cell-protease assay. Commun Biol. 2022;5:169.
[170] Sutanto F, Shaabani S, Oerlemans R, et al. Combining high-throughput synthesis and highthroughput protein crystallography for accelerated Hit identification. Angew Chem Int Ed.2021;60:18231–18239.
[171] Deetanya P, Hengphasatporn K, Wilasluck P, et al. Interaction of 8-anilinonaphthalene-1-sulfonate with SARS-CoV-2 main protease and its application as a fluorescent probe for inhibitor identification. Comput Struct Biotechnol J. 2021;19:3364–3371.
[172] Moghadasi SA, Esler MA, Otsuka Y, et al. Gain-of-signal assays for probing inhibition of SARS-CoV-2 Mpro/3CLpro in living cells. MBio. 2022;13:e0078422.
[173] Tomasello G,Armenia I,Molla G. The protein imager: a full-featured onlinemolecular viewer interface with server-side HQ-rendering capabilities. Bioinformatics. 2020;36:2909–2911.
[174] Omasits U, Ahrens CH, Müller S, et al. Protter: interactive protein feature visualization and integration with experimental proteomic data. Bioinformatics. 2014;30:884–886