This blog post is pulished also as a citeable article: doi.org/10.1080/0889311X.2024.2325352
Abstract
Following infection, SARS-CoV-2’s leader protein nsp1 is the very first viral protein to be expressed. Its sequence is highly conserved among different SARS-CoV-2 strains, indicating its vital function that makes it a promising target for drugs and vaccination. Nsp1 takes over the host cell protein expression machinery to facilitate the production of SARS-CoV-2 virions by the host cell by binding to the ribosome and obstructing the expression of any but the viral proteins. To date, 28 structures obtained by cryo-electron microscopy and X-ray diffraction have been published, showing the protein’s structural features as well as a number of interactions with host ribosomes. Nsp1 has also been shown to interfere with immune response pathways and is connected to the cytokine storm causing organ damage and failure in COVID-19 patients.
Introduction
SARS-CoV-2’s non-structural protein 1 (nsp1) is known by several names, including leader protein [1], host translation inhibitor [2] and host shutoff factor [3]. It is found in all beta-coronaviruses [4], and despite being relatively small with a length of only 180 amino acid residues [5], it plays a central role in the viral life cycle: nsp1 inhibits the host cell translation while facilitating viral translation [6]. Nsp1 is translated from the 5′ end of the SARS-CoV-2 genome [3] and is located in the beginning section of open reading frames 1a and 1ab (ORF1a) of the coronavirus genome; hence, it is the very first protein that is expressed by an infected host cell [7]. In the resulting polyprotein chain, nsp1 is located at the N-terminus.
Translation of the viral RNA into proteins occurs either shortly after the virus enters the host cell or after viral RNA replication inside the infected cell. The virus does not have its own proteins for RNA translation; instead, it uses the already existing translation machinery of the host cell: ribosomes [8]. They consist of several strands of ribosomal RNA (rRNA) and ribosomal proteins, which then form a larger (60S) and a smaller (40S) subunit [9]. The SARS-CoV-2 leader protein hijacks ribosomes and uses them for the replication of viral RNA, while the translation of original host cell proteins is suppressed [6]. In order to achieve this, nsp1 binds to the mRNA entrance channel of the ribosome [3] and facilitates the degradation of host-innate mRNA, leading, among other things, to severe inhibition of the cellular immune response which facilitates infection.
BLAST analysis [10] indicates that the sequence of nsp1 in SARS-CoV-1 and SARS-CoV-2 is unique, with no significant similarity to any known sequences in other viruses. The closest similarity in sequence found in vertebrate genes (with BLAST E values of no better than 0.85) [11]. Even though more recent studies indicated a common origin of alpha- and betacoronaviral nsp1, despite a lack of sequence homology, the mechanism of interaction between nsp1 and the host-cell-innate protein expression machinery is still considered specific to SARS-CoV-1 and SARS-CoV-2 [12].
The first full-length structure of SARS-CoV-1 nsp1 was obtained by nuclear magnetic resonance (NMR) in December 2006 (PDB entry: 2HSX) [13]. It would remain the only experimental structure of coronavirus nsp1 until February 2013 when in a crystallographic study, nsp1 from transmissible gastroenteritis virus (TGEV), an alphacoronavirus, was compared to the SARS-CoV-1 protein structure and functionality. TGEV nsp1, while exhibiting very little sequence homology to SARS-CoV-1 nsp1, contains the same structural features: a barrel fold made up of six β-strands with an α-helix located on one of its openings. Despite these similarities, it was postulated that both proteins inhibit host-innate translation by different mechanisms [14]. Five years later, the first full-length protein structure of an nsp1 was published, this time stemming from another alphacoronavirus, porcine epidemic diarrhoea virus (PEDV). This structure (PDB entry: 5XBC) again also showed similar structural features but low sequence homology [15].
In this review, we will describe the role and functions of this protein, with a particular focus on the available molecular structures.
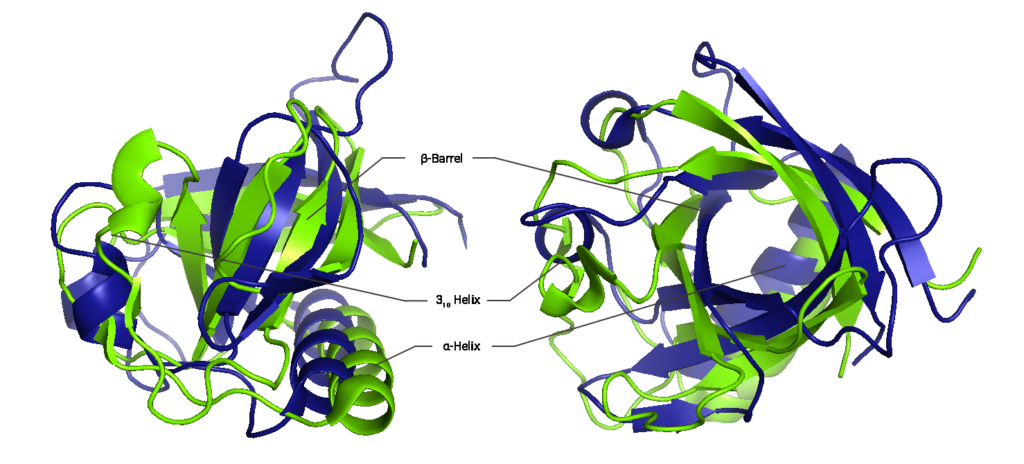
Figure 1. Overlay of the SARS-CoV-2 nsp1 N-terminal domain (PDB entry 7K7P; blue/darker color) and TGEV nsp1 (PDB entry: 6IVC; green/lighter color) with labels indicating common structural features.
Structural overview
The structure of nsp1 can be divided into two folded domains (Figure 2): an N-terminal domain (amino acid residues 1 to 128) and the smaller C-terminal domain (residues 148 to 180), with a flexible unstructured linker between them [3].
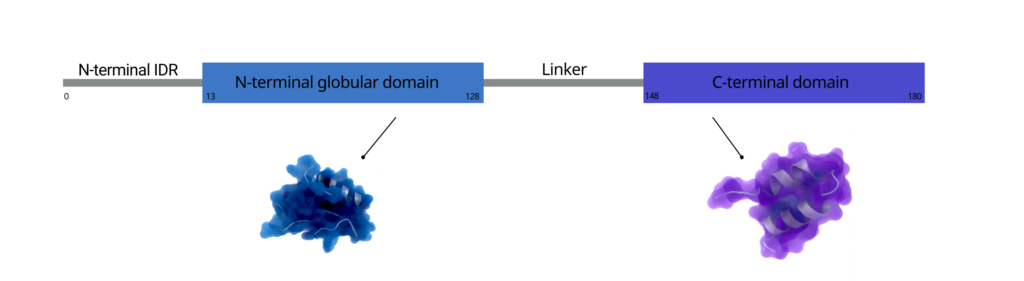
Figure 2. Domains of SARS-CoV-2 nsp1 in sequence with domain folds below. The protein consists of two ordered domains, the N-terminal globular domain (blue, left, PDB entry: 7EQ4) and the C-terminal domain (purple, right, PDB entry: 6ZOJ), with intrinsically disordered regions (N-terminal IDR) on the N-terminal end and between the two domains (Linker).
By the end of 2021, a total of 17 structures of the SARS-CoV-2 nsp1 were available (Table 1), of which only three were obtained by X-ray crystallography. These three structures only show the globular N-terminal domain with a resolution of up to 1.25 Å (PDB entry: 7EQ4). They are very similar in fold, with e.g. 7K7P and 7K3N exhibiting an RMSD of 0.35 Å based on Cα positions. The remaining 14 structures were obtained by cryo-electron microscopy (cryo-EM) and show the C-terminal domain of nsp1 in different complexes with ribosomal proteins and rRNA ranging in resolutions from 2.6 to 3.3 Å. Model-based predictions of the full-length structure of SARS-CoV-2 nsp1 indicate strong structural similarity to SARS-CoV-1 nsp1 (84 % sequence identity in the nsp1 sections of ORF1ab [16,17]) and hence a similar fold and function (see Figure 3) [12]. A near-complete assignment of the backbone chemical shifts obtained from 1H, 13C and 15N NMR experiments further supports this hypothesis by taking experimental data from various sources and methods into account [18].
Overview of available structures for SARS-CoV-1 and SARS-CoV-2 nsp1
SARS-CoV-1 | |||
---|---|---|---|
PDB ID | Method | Resolution [Å] | Description |
2GDT & 2HSX | NMR | – | First structure of its kind (amino acids 12 to 127), showing a previously unknown β-barrel fold. Deposited as an ensemble, (2GDT) as a consensus structure (2HSX) [Citation13]. |
7OPL | Cryo-EM | 4.12 | Nsp1 (amino acids 13 to 127) in complex with DNA polymerase α [Citation36]. |
SARS-CoV-2 | |||
PDB ID | Method | Resolution [Å] | Description |
6ZLW | Cryo-EM | 2.6 | C-terminal domain of nsp1 (amino acids 148 to 180) in complex with human 40S ribosomal subunit [Citation6]. |
6ZM7 | Cryo-EM | 2.7 | C-terminal domain of nsp1 (amino acids 152 to 180) in complex with human CCDC124–80S–EBP1 ribosomal complex [Citation6]. |
6ZME | Cryo-EM | 3.0 | C-terminal domain of nsp1 (amino acids 152 to 180) in complex with human CCDC124–80S–eERF1 ribosomal complex and rRNA [Citation6]. |
6ZMI | Cryo-EM | 2.6 | C-terminal domain of nsp1 (amino acids 148 to 180) in complex with human LYAR–80S ribosomal complex [Citation6]. |
6ZMO | Cryo-EM | 3.1 | C-terminal domain of nsp1 (amino acids 148 to 180) in complex with human LYAR–80S–eEF1a ribosomal complex and rRNA [Citation6]. |
6ZMT | Cryo-EM | 3.0 | C-terminal domain of nsp1 (amino acids 152 to 180) in complex with human pre-40S-like ribosome and rRNA, state 1 [Citation6]. |
6ZN5 | Cryo-EM | 3.2 | C-terminal domain of nsp1 (amino acids 152 to 180) in complex with human pre-40S-like ribosome and rRNA, state 2 [Citation6]. |
6ZOJ | Cryo-EM | 2.8 | C-terminal domain of nsp1 (amino acids 148 to 180) in complex with human 40S ribosomal subunit [Citation3]. |
6ZOK | Cryo-EM | 2.8 | C-terminal domain of nsp1 (amino acids 148 to 180) in complex with human 40S ribosomal subunit body domain and 18S rRNA [Citation3]. |
6ZOL | Cryo-EM | 2.8 | Human 40S ribosomal subunit head domain and 18S rRNA (no involvement of nsp1) [Citation3]. |
6ZON | Cryo-EM | 3.0 | C-terminal domain of nsp1 (amino acids 151 to 180) in complex with human 43S preinitiation ribosome and 18S rRNA, state 1 [Citation6]. |
6ZP4 | Cryo-EM | 2.9 | C-terminal domain of nsp1 (amino acids 148 to 180) in complex with human 43S preinitiation ribosome and 18S rRNA, state 2 [Citation6]. |
7EQ4 | X-ray Diffraction | 1.25 | Globular N-terminal domain (amino acids 11 to 125) [Citation40]. |
7JQB | Cryo-EM | 2.7 | C-terminal domain of nsp1 (amino acids 145 to 180) in complex with rabbit 40S ribosomal subunit and rRNA [Citation7]. |
7JQC | Cryo-EM | 3.3 | C-terminal domain of nsp1 (amino acids 145 to 180) in complex with rabbit 40S ribosomal subunit and CrPV IRES [Citation7]. |
7K3N | X-ray Diffraction | 1.65 | N-terminal globular domain (amino acids 13 to 127) [Citation41]. |
7K5I | Cryo-EM | 2.9 | C-terminal domain of nsp1 in complex with human 40S ribosomal subunit and 18s rRNA [Citation23]. |
7K7P | X-ray Diffraction | 1.77 | N-terminal globular domain (amino acids 10 to 127) [Citation16]. |
8A4Y | X-ray Diffraction | 1.099 | N-terminal domain (amino acids 10 to 126) in complex with N-(2,3-dihydro-1H-inden-5-yl)acetamide [Citation37]. |
8A55 | X-ray Diffraction | 0.99 | N-terminal globular domain (amino acids 10 to 126) [Citation17]. |
8AOU | NMR | – | Full-length structure (amino acids 3 to 182) [Citation21]. |
8ASQ | X-ray Diffraction | 1.15 | N-terminal globular domain (amino acids 10 to 126) in complex with N-methyl-1-(4-(thiophen-2-yl)phenyl)meth-anamine [Citation17]. |
8AYS | X-ray Diffraction | 1.37 | N-terminal globular domain (amino acids 10 to 126) in complex with 4-(2-aminothiazol-4-yl)phenol [Citation17]. |
8AYW | X-ray Diffraction | 1.10 | N-terminal globular domain (amino acids 10 to 126) in complex with (S)-1-(4-chlorophenyl)ethan-1-amine [Citation17]. |
8AZ8 | X-ray Diffraction | 1.18 | N-terminal globular domain (amino acids 10 to 126) in complex with 2-(benzylamino)ethan-1-ol [Citation17]. |
8AZ9 | X-ray Diffraction | 1.42 | N-terminal globular domain (amino acids 10 to 126) in complex with 1-(2-(3-chlorophenyl)thiazol-4-yl)-N-methyl-methanamine [Citation17]. |
8CRF | X-ray Diffraction | 1.15 | N-terminal globular domain (amino acids 10 to 126) in complex with fragment hit 5E11 [Citation42]. |
8CRK | X-ray Diffraction | 1.1 | N-terminal globular domain (amino acids 10 to 126) in complex with fragment hit 7H2 [Citation42]. |
8CRM | X-ray Diffraction | 1.42 | N-terminal globular domain(amino acids 10 to 126) in complex with fragment hit 11C6 [Citation42]. |
The structural models of the N-terminal domain of nsp1 derived from X-ray diffraction have been evaluated by the Coronavirus Structural Task Force [19]. The difference Fourier map for PDB entry 7EQ4 contain a total of nine peaks larger than 5σ and a methionine residue which should be flipped to better fit the electron density map. 7K3N contains only four peaks above 5σ, but the solvent molecules are not modelled ideally. 7K7P has five peaks above 5σ, an alternative confirmation for the residue His74 in chain B might improve the structure model.
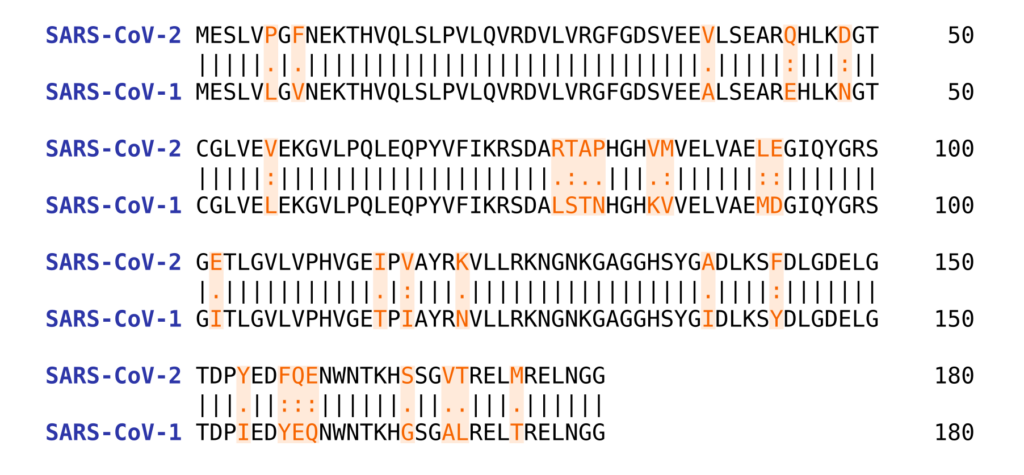
Figure 3. Sequence alignment of SARS-CoV-1 and SARS-CoV-2 nsp1, with differences in sequence highlighted.
Complementary data on the protein’s secondary structure features were obtained via 1H-, 13C- and 15N-NMR in solution and published in May 2021, revealing details about the Cβ positions of the full-length apo structure and indicating intramolecular interactions between the two ordered domains [20].
A full-length structure of SARS-CoV-2 nsp1 with atomic resolution (0.99 Å, PDB entry: 8A55) was reported in October 2022 by Shumeng et al. [17]. The group has also succeeded in crystallizing the N-terminal domain (amino acids 10 to 126) using common standardized crystallization screens, resulting in three types of crystals with space group P43212.
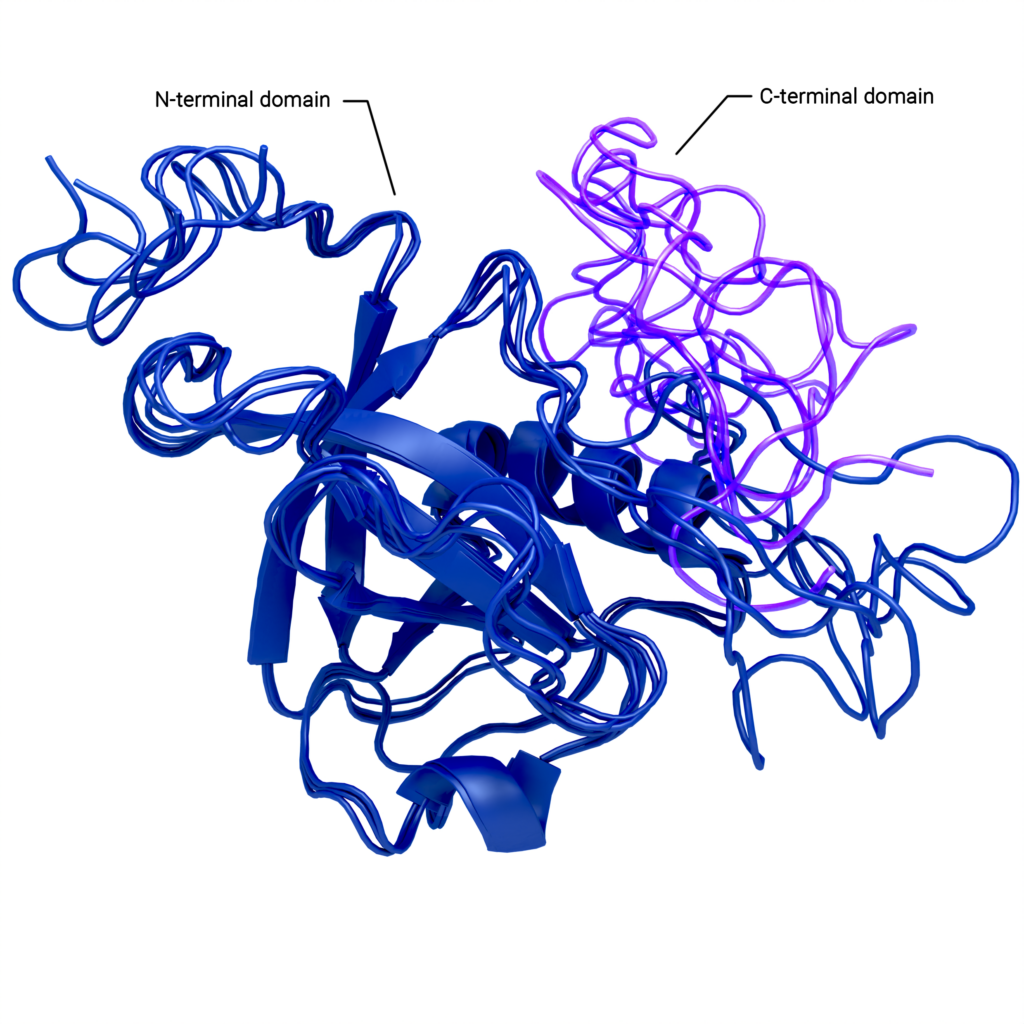
Figure 4. NMR ensemble of SARS-CoV-2 nsp1 in solution, showing a subset of four structures from a structural ensemble (PDB entry: 8AOU). The disordered C-terminal domain (purple) shows affinity towards the N-terminal domain’s (blue) face containing the α-helix (α1) that blocks one side of the β-sheet barrel.
A complete NMR structure model of both domains was reported in January 2023 by Wang et al. [21]. It shows that the C-terminal domain, which is structurally disordered in free nsp1, interacts with the same region of the N-terminal domain that shows affinity towards viral mRNA when the protein is bound to a ribosome.
Structural features
The N-terminal globular domain comprises residues 13 to 128 and is made up of six β-strands forming a mixed parallel/anti-parallel barrel and an α-helix covering one of its openings (Figure 5), with a short 310 helix (η1) next to the barrel. The globular domain’s sequential arrangement is β1-α1-β2-η1-β3-η3-β4-β5-β6, the sequence of strands in the barrel is β1-β2-β5-β3-β4-β6(-β1). The protein’s N- and C-terminal loops, residues 1 to 12 and 129 to 147 respectively, are flexibly disordered [13]. A recent study reports an additional flexible 310 helix (η3) located between β3 and β4, comprising amino acids 80 to 83 [17].
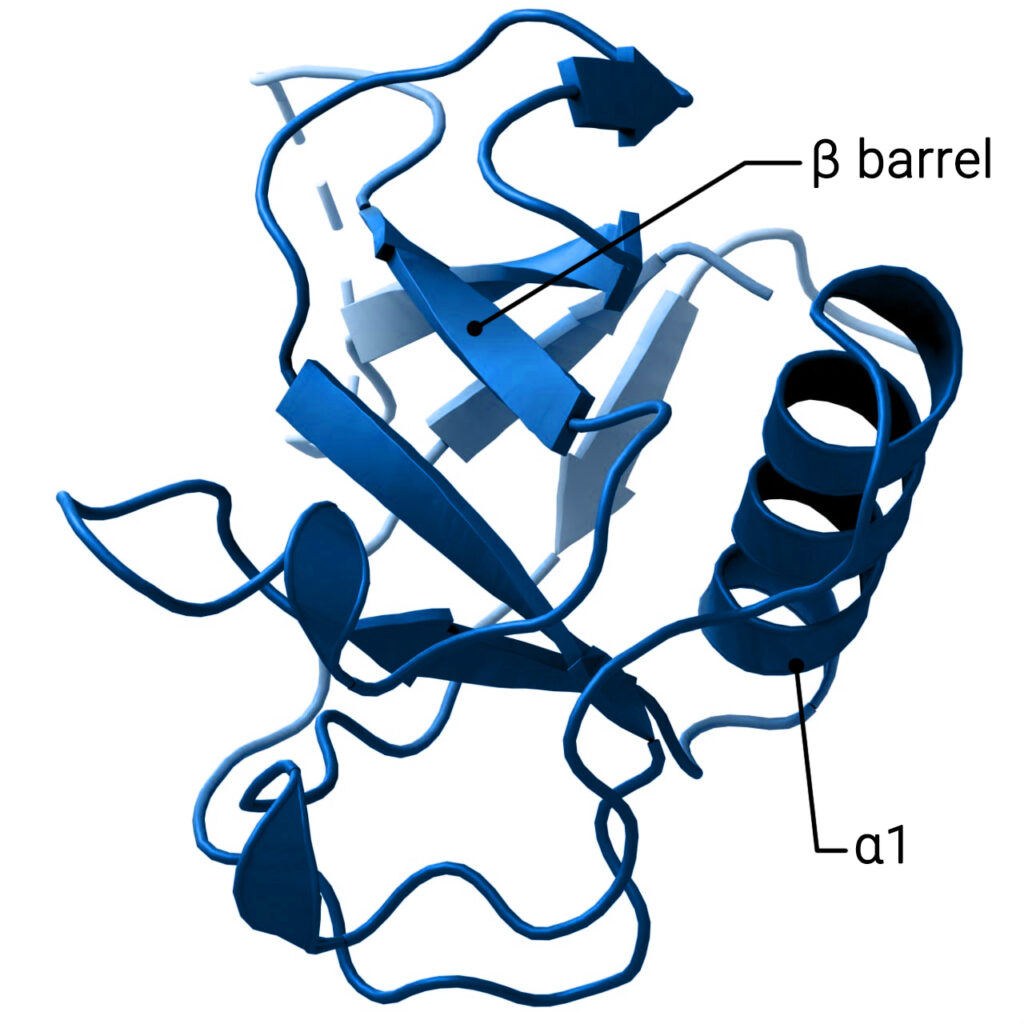
Figure 5. N-terminal globular domain of SARS-CoV-2 nsp1 (PDB entry: 7EQ4). The β-sheets form a semi-open barrel structure, with one aperture blocked by an α-helix (α1).
The C-terminal domain (residues 148 to 180) containing the two helices α2 (residues 153 to 159) and α3 (residues 166 to 178), which are responsible for anchoring the protein to the ribosome [22]. This fundamental interaction will be described in the following section.
The two domains are connected by a disordered 20 residues long linker (residues 128 to 147), which is usually omitted in structural studies.
Interaction with ribosomes
The C-terminus of Nsp1 binds to the 40S subunit of the host ribosome. This inhibition causes major disruption to the cellular metabolism and disables important immune response pathways [7].
Human ribosomes, also known as 80S ribosomes (the number indicates their sedimentation coefficient), consist of two subunits referred to as 40S and 60S. Once nsp1 is released into the cytoplasm of an infected cell, its C-terminus interacts with the 40S ribosomal subunit in different ways, forming various complexes that might involve additional molecular components. Complexes can form under involvement of TSR1, a biogenesis factor present in the cytosol, or various eukaryotic initiation factors such as eIF1, eIF1A, eIF3 and an eIF2–tRNAi–GTP (guanosine triphosphate) complex, effectively constituting 43S preinitiation complexes (PIC) [6].
Complexes formed between nsp1’s C-terminus and complete 80S ribosomes might also involve additional components, including CCDC124 (coiled-coil domain containing short open reading frame 124), ABCE1 (an enzyme relevant for ribosome recycling and ATP cleavage), eRF1 (a translation termination factor), or the LYAR (Ly 1 antibody reactive) protein, as well as an eEF1A–GTP–tRNA complex. All of these 80S complexes are translationally inactive, and some interactions have not been observed in an nsp1-free environment before [6]. The binding mechanism between nsp1 and the ribosomal subunit is identical in either case:
Nsp1’s C-terminal helix α2 interacts hydrophobically with the ribosomal protein uS5 of the 40S body domain and electrostatically with ribosomal protein uS3 of the 40S head domain, placing nsp1 inside the ribosome’s mRNA entry channel and locking the head domain in a conformational position that prevents loading of mRNA, hence rendering translation impossible. A KH motif (residues 164 and 165) in the loop following α2 in nsp1’s C-terminal sequence can subsequently interact with the ribosome’s nucleotide pseudoknot structure h18 on the rRNA (Figure 6). The helix α3 in nsp1’s C-terminal domain forms additional stabilizing interactions with h18 and uS5 [6]. While nsp1 exhibits no affinity towards 60S ribosomal subunits, its affinity to the 40S subunit, and therefore the entire 80S ribosome, relies on the KH motif in its C-terminal domain. It could be shown that mutations in these positions disrupt the colocalization of nsp1 with both 40S subunits and 80S ribosomes in human embryonic kidney (HEK) cells. To confirm this, Thomes et al. showed experimentally that K164A and H165A mutants of both SARS-CoV-1 and SARS-CoV-2 showed no binding affinity towards 40S ribosomal subunits, unlike their non-mutated variants [6].
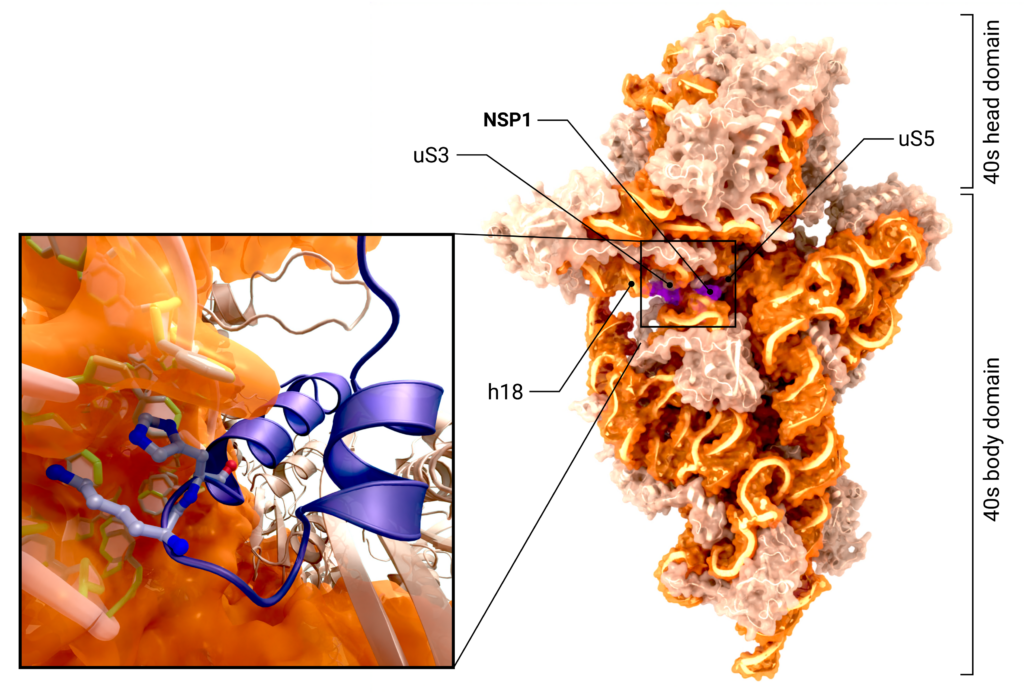
Figure 6. Interaction between SARS-CoV-2 nsp1 (purple), human ribosomal RNA (bright orange) and ribosomal proteins (mainly 40S, dark orange), with a highlight on nsp1’s KH motif (molecular representation in the zoomed-in view) mediating the interaction (PDB entry: 6ZOJ).
Nsp1 sterically hinders the physiological conformation that is necessary for the ribosome to be able to load the host cell’s mRNA by limiting the rotational movement of the head domain of the 40S subunit. Due to this blockade, nsp1 plays an important role in suppressing the host’s immune response by preventing expression of proteins involved in immune pathways, such as cytokines and interferons. SARS-CoV-2’s own viral RNA is capable of overcoming the blockade. For this, various mechanisms via specific stem–loop structures in the viral RNA’s 5′ untranslated region (UTR) are being discussed [1], possibly involving the host-innate essential initiation factor eIF3 [6,7]. In a preprint from Shi et al., the viral 5′ UTR is suggested to interact with the C-terminal domain of nsp1 in a way that renders simultaneous interaction with the ribosome sterically impossible and therefore lifts the blockade for the viral mRNA [23]. It has also been suggested that while not all ribosomes are blocked by nsp1, the remaining are five times more likely to translate mRNA including the viral RNA’s 5′ untranslated region, causing translation of viral RNA to be significantly more efficient [3].
Complementary knowledge
Similarity to SARS-CoV-1
SARS-CoV-2 nsp1 is highly homologous to SARS-CoV-1 nsp1 both in sequence (84% identity) and structure. However, there are a number of structural differences between the two proteins that may slightly alter their function in the context of their respective viral life cycles. For instance, residues 23 to 25, a sequence that is present in both SARS-CoV-1 and SARS-CoV-2 nsp1, folds as a 310 helix (η1) in SARS-CoV-2, but not in SARS-CoV-1. Without any sequential differences in the immediate vicinity that could stabilize this structural feature, it is supported via a new interaction with residue Q63 on η2 (η1 in SARS-CoV-1, residues 63 to 65) [16].
Furthermore, SARS-CoV-2’s β4-strand (residues 84 to 92) contains four additional amino acids due to adjacent mutations K84V, V85M, and M92L. This extension allows for auxiliary interactions involving the loops after β3 and β4, causing significant mean displacement of their Cα (16.6 Å and 27.6 Å, respectively). This new network of polar interactions stabilizes an additional short β-strand not present in SARS-CoV-1 (β5; residues 95 to 97). Similarly, β1 (residues 13 to 20), β3 (residues 68 to 73) and β6 (β5 in SARS-CoV-1, residues 103 to 109 in SARS-CoV-2) have been extended by one amino acid each as well. Three mutations—A38V, E44Q and N48D—can be found on the structurally conserved α1-helix (residues 34 to 49), the strands β2 (residues 51 to 54) and β7 (β6 in SARS-CoV-1; residues 117 to 123 in SARS-CoV-2) are conserved as well [16]. An NMR study on nsp1 secondary structure, on the other hand, reports slightly different amino acid ranges for many of these features and did not find evidence for the SARS-CoV-2-specific β5-strand observed via X-ray crystallography, defining the sequence as part of a dynamic region instead, implying that the observed differences are due to crystal packing [20].
Similarity between different SARS-CoV-2 strains
Sequence alignment analysis across different SARS-CoV-2 strains shows that nsp1 mutates rarely, with only one single mutation (D75E) evident on the C-terminal side of the β4 sheet that is unlikely to significantly affect the protein’s functionality due to the conserved polarity between aspartic and glutamic acid [22]. This lack of endemic mutants hints at nsp1’s crucial function in the viral life cycle.
Cleavage of host-innate mRNA
In addition to blocking host mRNA translation at ribosomes, the 40S–nsp1 complex causes cell-innate capped mRNA to degrade by inducing endonucleolytic cleavage in the untranslatable region (UTR) near its 5′ terminus. However, nsp1 does not exhibit RNase activity itself but is believed to employ a host-innate Rnase, although it is unclear which one that may be [24]. SARS-CoV-2’s viral RNA itself is not targeted by this degradation process [1], leading to a rise in viral RNA activity compared to host-innate RNA activity over time. A leader sequence present in all SARS-CoV-2 RNA strands in the UTR at the 5′ terminus is thought to protect against this host cell enzymatic activity. To this end, a section on the N-terminal domain of ribosome-bound nsp1 that is blocked by the C-terminal domain in the free protein interacts with the 5′ UTR on the viral RNA [21]. Hence, the viral RNA is blocked from interacting with the endonucleolytically active nsp1–40S complex and is instead abundantly available for translation by ribosomes free of nsp1 interference. At the same time, cell-innate mRNA readily interacts with the complex and is gradually deactivated, until it is unable to compete with the significantly more abundant viral mRNA for functional ribosomes [3,24].
Immune response interferences
Nsp1 has not only been shown to facilitate the degradation of host-innate mRNA—it also interferes with its nuclear export. By binding to the transcriptional repressor NFX1, an important agent in nuclear transcription, nsp1 appears to not immediately limit its ability to bind RNA, but rather change the conformation of NFX1–RNA complexes to a state that is unable to dock with export adapters that allow the mRNA to permeate the nuclear pore complex, as indicated by a series of in vitro glutathione S-transferase pull-down assays [25]. Such an effect has also been observed for SARS-CoV-1 [26] and other viruses, for example influenza via its NS1 protein [27]. Inhibiting the export of newly transcribed mRNA out of the nucleus severely limits the capability of the infected cell to adjust their transcriptome to the viral threat and prevents the expression of proteins that would be crucial for a functional immune response, such as type-I interferons [28,29]. At the same time, it contributes to the reduction of host-innate mRNA activity in the cytoplasm and hence creates a favourable environment for viral reproduction. The structural mechanism of this inhibition remains to be investigated.
Interestingly, another study suggests that expression of certain proteins involved in immune response are in fact upregulated in cells infected with SARS-CoV-2 [30], especially proinflammatory cytokines e.g. IL-22, an interleukin protein involved in tissue repair processes that has been shown to play an important role in maintaining the integrity of epithelial cells [31]. This effect is observed in severe cases of COVID-19 that require hospitalization and intensive care treatment [32], as increased secretion of these hyperinflammatory factors causes various detrimental complications in patients, among them liver damage, heart and kidney failure [33]. How their expression avoids nsp1-mediated downregulation remains unclear.
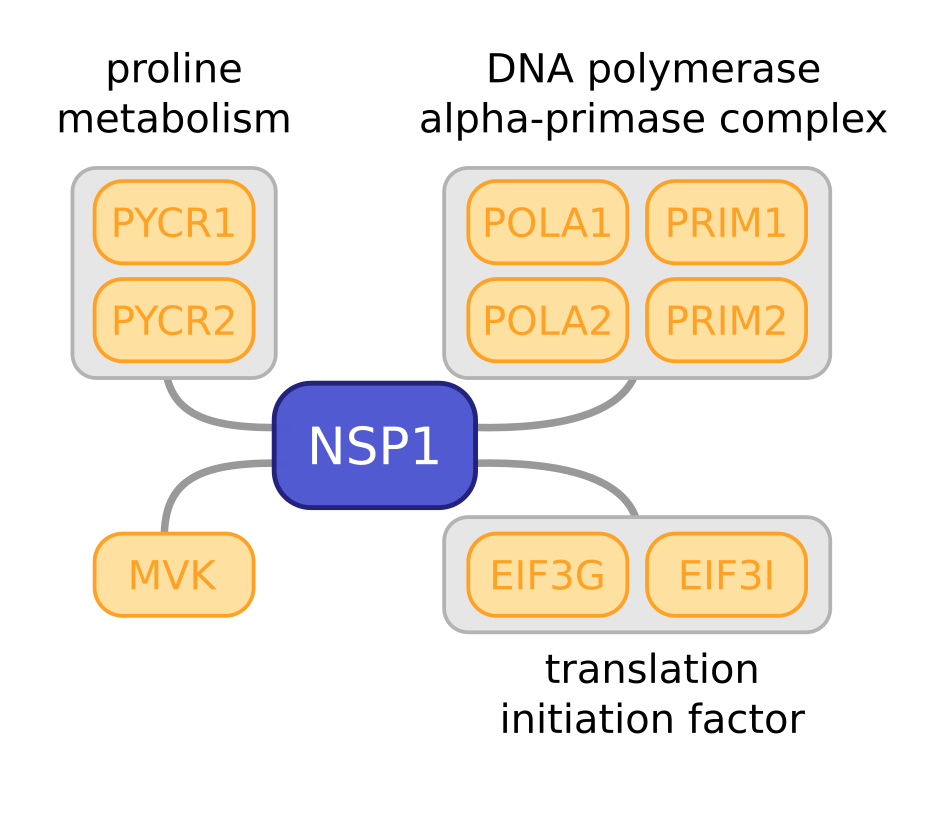
Figure 7. Interactome of nsp1 with host cell factors, grouped by function: Pyrroline-5-carboxylate reductase 1 and 2 (PYCR1 & PYCR2), mevalonate kinase (MVK), DNA polymerase alpha subunits 1 and 2 (POLA1 & POLA2), DNA primase small and large subunits (PRIM1 & PRIM2) as well as Eukaryotic translation initiation factor 3 subunits G and I (EIF3G & EIF3I).
A comprehensive study on the human cell interactome of SARS-CoV-2 employing tandem affinity purification and proximity labelling very likely indicates an interaction between the nsp1 N-terminal globular domain and the DNA polymerase α complex (Pol α) (see Figure 7). This interaction can also be observed for SARS-CoV-1 nsp1 [34], but not for any nsp1 homolog in other coronaviruses, hinting at an interaction mechanism that is unique to SARS-CoV-1 and SARS-CoV-2 [35]. These results were later confirmed experimentally by cryo-EM on the basis of SARS-CoV-1 nsp1, as it showed a somewhat higher affinity towards the Pol α [36]. The implications of these interactions for SARS-CoV-2 virulence remains to be investigated.
Therapeutic interest in nsp1
The leader protein nsp1 is a crucial factor for pathogenicity shared by alpha- and betacoronaviruses. In spite of the fact that little sequential conservation is evident between nsp1 variants in different sarbecovirus genera, their function in the infection cycle is similar, namely, inhibiting the host cell immune response and facilitating expression of viral proteins. Disabling nsp1 activity is therefore a promising strategy for COVID-19 therapy. In addition, only very few mutations have been identified in the SARS-CoV-2 nsp1 sequence across viral strains [12]. Hence, the leader protein is a promising drug target that is unlikely to escape therapeutic approaches by mutation.
A virtual screening of potential drug molecules assessing their stability in complex with nsp1 by molecular dynamics (MD) simulations identified a total of 16 compounds with a high affinity independent of protein conformation. Three molecules stood out with the most favourable energy scores (ranging from ‒6.9 to ‒10.4 kcal mol‒1), namely tirilazad (DB13050), phthalocyanine (DB12983) and Zk-806450 (DB2112). These three even exceeded the calculated binding affinity of alisporivir and cyclosporine, two compounds that have previously been identified as potent nsp1 inhibitors in SARS-CoV-1. The study concludes that the specific binding pockets for these drug molecules in SARS-CoV-2 nsp1, which are all located in the protein’s C-terminal domain, do not naturally occur in the human proteome, making severe side effects of medication with the compounds unlikely. However, inferences of this in silico investigation for actual biological systems are so far speculative, necessitating further analysis in vitro. [4]
Another molecular dynamics study screened more than 5,000 phytochemicals traditionally used in drug development for the stability of their interaction with the inter-motif loop (K164 and H165) in nsp1’s C-terminal domain that is responsible for the protein’s ribosomal affinity. Additionally, density functional theory (DFT) calculations were performed to support the results. The five most stable complexes (with energy scores ranging from ‒9.63 to ‒8.75 kcal mol‒1) were formed with the compounds dihydromyricetin, 10-demethylcephaeline, dihydroquercetin, pseudolycorine and tricetin. All of these molecules are currently being assessed as drugs for various diseases, including diabetes mellitus, hepatitis and cancer. This may speed up the preparation required for clinical studies in the context of COVID-19 [22].
A recent screening study identified two binding sites on the N-terminal domain of nsp1 for a total of five ligand fragments containing phenyl rings alongside, in three cases, heterocyclic five-rings [17]. The first binding site identified in the study consists of β1, β7 and several amino acids from α1, while the second, more shallow binding site is located between α1, β6, β7 and η1. The screening was performed on nsp110–126 crystals, interactions between nsp1 N-terminal domain and the ligands involved additional copies of the protein (PDB entries: 8AZ9, 8AYS, 8ASQ, 8AZ8 and 8AYW). The binding pockets are not found in SARS-CoV-1 and MERS [17].
Another study reports a total of four putative binding sites on the surface of nsp1’s N-terminal domain, a result obtained from an analysis based on unbiased MD simulation [37]. Crystallographic experiments were unable to confirm these findings, as the binding sites were at least partially blocked by crystal packing.
Nsp1’s interaction with the 5′ untranslated region of viral mRNA could be changed to obtain an attenuated live vaccine [1]. Studies to create such an attenuated viral strain by purposeful deletions in nsp1’s sequence have been successfully conducted with the protein’s MHV (mouse hepatitis virus) homologue [38].
Summary
Leader protein nsp1 is encoded at the 5′ terminus of ORF1a of the SARS-CoV-2 genome and is the first viral protein to be expressed by an infected host cell [7]. The relatively short protein is present in a subset of betacoronaviruses, and even though a protein with a similar secondary structure fulfilling similar roles is part of the proteome of certain alphacoronaviruses—hence also called nsp1—, the sequences of the two proteins called nsp1 show very little homology (for instance, porcine epidemic diarrhea virus PEDV and SARS-CoV-1 nsp1 share a sequence identity of only 12%) [12]. As an important virulence and pathogenicity factor, nsp1 is responsible for abolishing the host’s protein biosynthesis by deactivating ribosomes and inducing endonucleolytic cleavage of capped mRNA. SARS-CoV-2’s own viral mRNA is unsusceptible to these cleavage events facilitated by the nsp1–40S complex. Thereby, nsp1 affects the host cell’s transcriptome and promotes the expression of viral components while at the same time downregulating all other processes involving protein synthesis, including various immune response pathways [1].
Discussion & outlook
With its critical role of inhibiting host-innate protein expression while simultaneously facilitating translation of the viral genome, nsp1 is very important for the SARS-CoV-2 viral infection cycle. Despite being a rather small protein comprising only 180 amino acids in two distinct domains, its full-length structure has not yet been solved. This is due to the C-terminal domain being primarily disordered in absence of a binding partner, and its mechanisms of interaction with the host cell translation machinery not yet completely being understood [12]. Nonetheless, recent studies offer insights into possible interactions between nsp1 and the human ribosome and shed light on potential mechanisms they are involved in [21].Studies have shown that nsp1 is responsible for inhibition of major immune response pathways, downregulating expression of several interferons and cytokines. It has also been suggested to promote expression of certain immune response factors, hence supporting the cytokine storm that is responsible for cases of organ failures. leading to many COVID-19 fatalities [39]. Understanding these processes on a molecular level is crucial to prevent life-threatening cytokine storms in COVID-19 patients [33].
The characteristic structure of nsp1 allows it to engage in very specific interactions that are indispensable for the viral life cycle [6]. Its sequence is highly conserved between different strands of SARS-CoV-2, exhibiting a remarkably low mutation rate [22]. Even though not all mechanisms of the protein are fully understood, these properties make it a promising drug target, allowing for precise inhibition of crucial virulent processes with low risk of severe side effects or drug evasion via mutation.
In silico screenings have identified a number of compounds that show favourable binding affinities to nsp1 and, hence, come into consideration as potential inhibitors. However, further investigation on actual biological systems in vitro or in vivo is necessary [4].
Remaining questions
SARS-CoV-2 leader protein nsp1 is known to interfere with various important physiological processes in infected host cells on an immediate molecular level, including gene expression at the nucleus [25], ribosomal RNA translation [7] and enzymatic degradation of host-innate mRNA [24]. Additionally, it has affinity towards ribosomes and nuclear transcription agents, as well as the DNA polymerase α complex [36]. While all of these interactions contribute to the virulence of SARS-CoV-2, it remains unclear which is the most crucial factor. Structural data exist for a number of ribosomal complexes [6], however, the mechanisms and implications of these processes remain mostly speculative. As similar interactions have been observed for other disease-causing viruses including [26], but not limited to other coronaviruses [27], thorough investigation of these processes promises to enable insights even beyond the scope of the immediate threat of the COVID-19 pandemic.
Acknowledgements
This work was supported by the German Federal Ministry of Education and Research under Grant 05K19WWA and 05K22GU5; and Deutsche Forschungsgemeinschaft under Grant TH2135/2-1. The authors would also like to thank Rosemary Wilson for support and discussion and Gianluca Tomasello for his assistance with the reviews’ illustrations. All figures are courtesy of the Coronavirus Structural Task Force, which retains copyright for both the text and the figures.
References
[1] Vankadari N, Jeyasankar NN, Lopes WJ. Structure of the SARS-CoV-2 Nsp1/5-untranslated
region complex and implications for potential therapeutic targets, a vaccine, and virulence. J
Phys Chem Lett. 2020;11:9659–9668. doi:10.1021/acs.jpclett.0c02818
[2] Anwar MU, Adnan F, Abro A, et al. Combined deep learning and molecular docking
simulations approach identifies potentially effective FDA approved drugs for repurposing
against SARS-CoV-2. Comput Biol Med. 2020 [cited 2022 Apr 13];141:105049. doi:10.1016/j.
compbiomed.2021.105049. Available from: https://chemrxiv.org/engage/chemrxiv/articledetails/
60c74a96f96a007f8228747a.
[3] Schubert K, Karousis ED, Jomaa A, et al. SARS-CoV-2 Nsp1 binds the ribosomal mRNA
channel to inhibit translation. Nat StructMol Biol. 2020;27:959–966. doi:10.1038/s41594-020-
0511-8
[4] de Lima Menezes G, da Silva RA. Identification of potential drugs against SARS-CoV-2 nonstructural
protein 1 (nsp1). J Biomol Struct Dyn. 2021;39:5657–5667. doi:10.1080/07391102.
2020.1792992
[5] Yoshimoto FK. The proteins of severe acute respiratory syndrome coronavirus-2 (SARS CoV-2
or n-COV19), the cause of COVID-19. Protein J. 2020;39:198–216. doi:10.1007/s10930-020-
09901-4
[6] Thoms M, Buschauer R, Ameismeier M, et al. Structural basis for translational shutdown
and immune evasion by the Nsp1 protein of SARS-CoV-2. Science. 2020;369:1249–1255.
doi:10.1126/science.abc8665
[7] Yuan S, Peng L, Park JJ, et al. Nonstructural protein 1 of SARS-CoV-2 is a potent
pathogenicity factor redirecting host protein synthesis machinery toward viral RNA.Mol Cell.
2020;80:1055–1066.e6. doi:10.1016/j.molcel.2020.10.034
16 J. KAUB ET AL.
[8] Tirumalai MR, Rivas M, Tran Q, et al. The peptidyl transferase center: a window to the past.
Microbiol Mol Biol Rev. 2021; 85:e00104-21.
[9] Khatter H, Myasnikov AG, Natchiar SK, et al. Structure of the human 80S ribosome. Nature.
2015;520:640–645. doi:10.1038/nature14427
[10] Altschul SF, Madden TL, Schäffer AA, et al. Gapped BLAST and PSI-BLAST: a new
generation of protein database search programs. Nucleic Acids Res. 1997;25:3389–3402.
doi:10.1093/nar/25.17.3389
[11] Connor RF, Roper RL. Unique SARS-CoV protein nsp1: bioinformatics, biochemistry and
potential effects on virulence. TrendsMicrobiol. 2007;15:51–53. doi:10.1016/j.tim.2006.12.005
[12] MinY-Q,MoQ,Wang J, et al. SARS-CoV-2nsp1: bioinformatics, potential structural and functional
features, and implications for drug/vaccine designs. Front Microbiol. 2020;11:587317.
doi:10.3389/fmicb.2020.587317
[13] Almeida MS, Johnson MA, Herrmann T, et al. Novel β-barrel fold in the nuclear magnetic
resonance structure of the replicase nonstructural protein 1 from the severe acute respiratory
syndrome coronavirus. J Virol. 2007;81:3151–3161. doi:10.1128/JVI.01939-06
[14] Jansson AM. Structure of alphacoronavirus transmissible gastroenteritis virus nsp1 has
implications for coronavirus nsp1 function and evolution. J Virol. 2013;87:2949–2955.
doi:10.1128/JVI.03163-12
[15] Shen Z, Ye G,Deng F, et al. Structural basis for the inhibition of host gene expression by porcine
epidemic diarrhea virus nsp1. J Virol. 2018;92:e01896-17.
[16] Clark LK, Green TJ, Petit CM. Structure of nonstructural protein 1 fromSARS-CoV-2. J Virol.
2021;95:e02019-20.
[17] Ma S, Damfo S, Lou J, et al. Two ligand-binding sites on SARS-CoV-2 non-structural
protein 1 revealed by fragment-based X-ray screening. Int J Mol Sci. 2022;23:12448.
doi:10.3390/ijms232012448
[18] Wang Y, Kirkpatrick J, zur Lage S, et al. 1H, 13c, and 15N backbone chemical-shift assignments
of SARS-CoV-2 non-structural protein 1 (leader protein). Biomol NMR Assign.
2021;15:287–295. doi:10.1007/s12104-021-10019-6
[19] Croll T, Diederichs K, Fischer F, et al. Making the invisible enemy visible [Internet]. bioRxiv;
2020 [cited 2022 Jun 2]. Available from: https://www.biorxiv.org/content/10.11012020.10.07.
307546v2.
[20] Agback T, Dominguez F, Frolov I, et al. 1 H, 13 C and 15 N resonance assignment of the SARSCoV-
2 full-length nsp1 protein and its mutants reveals its unique secondary structure features
in solution. PLoS One. 2021;16(12):e0251834. doi:10.1371/journal.pone.0251834
[21] Wang Y, Kirkpatrick J, zur Lage S, et al. Structural insights into the activity regulation
of full-length non-structural protein 1 from SARS-CoV-2. Structure. 2023;31:128–137.e5.
doi:10.1016/j.str.2022.12.006
[22] PrabhuD, Rajamanikandan S, SureshanM, et al.Modelling studies reveal the importance of the
C-terminal intermotif loop of NSP1 as a promising target site for drug discovery and screening
of potential phytochemicals to combat SARS-CoV-2. JMol GraphicsModell. 2021;106:107920.
doi:10.1016/j.jmgm.2021.107920
[23] Shi M, Wang L, Fontana P, et al. SARS-CoV-2 Nsp1 suppresses host but not viral translation
through a bipartite mechanism[Internet]. Cell Biol. 2020 [cited 2022 Apr 13]. Available from:
http://biorxiv.org/lookup/doi/10.11012020.09.18.302901.
[24] Huang C, Lokugamage KG, Rozovics JM, et al. SARS coronavirus nsp1 protein induces
template-dependent endonucleolytic cleavage of mRNAs: viral mRNAs are resistant to nsp1-
induced RNAcleavage. PLoS Pathog. 2011;7:e1002433. doi:10.1371/journal.ppat.1002433
[25] Zhang K, Miorin L, Makio T, et al. Nsp1 protein of SARS-CoV-2 disrupts the mRNA export
machinery to inhibit host gene expression. Sci Adv. 2021;7:eabe7386. doi:10.1126/sciadv.
abe7386
[26] Narayanan K, Huang C, Lokugamage K, et al. Severe acute respiratory syndrome coronavirus
nsp1 suppresses host gene expression, including that of type I interferon, in infected cells. J
Virol. 2008;82:4471–4479. doi:10.1128/JVI.02472-07
CRYSTALLOGRAPHY REVIEWS 17
[27] York A, Fodor E. Biogenesis, assembly, and export of viralmessenger ribonucleoproteins in the
influenza A virus infected cell. RNA Biol. 2013;10:1274–1282. doi:10.4161/rna.25356
[28] Xia H, Cao Z, Xie X, et al. Evasion of type I interferon by SARS-CoV-2. Cell Rep.
2020;33:108234. doi:10.1016/j.celrep.2020.108234
[29] Lei X, Dong X, Ma R, et al. Activation and evasion of type I interferon responses by SARSCoV-2
[30] Liao Y, Li X, Mou T, et al. Distinct infection process of SARS-CoV-2 in human bronchial
epithelial cell lines. J Med Virol. 2020;92:2830–2838. doi:10.1002/jmv.26200
[31] Alcorn JF. IL-22 plays a critical role in maintaining epithelial integrity during pulmonary
infection. Front Immunol. 2020;11:1160. doi:10.3389/fimmu.2020.01160
[32] Costela-Ruiz VJ, Illescas-Montes R, Puerta-Puerta JM, et al. SARS-CoV-2 infection: the
role of cytokines in COVID-19 disease. Cytokine Growth Factor Rev. 2020;54:62–75.
doi:10.1016/j.cytogfr.2020.06.001
[33] Fara A, Mitrev Z, Rosalia RA, et al. Cytokine storm and COVID-19: a chronicle of proinflammatory
cytokines. Open Biol. 2020;10:200160. doi:10.1098/rsob.200160
[34] Gordon DE, Hiatt J, Bouhaddou M, et al. Comparative host-coronavirus protein interaction
networks reveal pan-viral disease mechanisms. Science. 2020;370:eabe9403. doi:10.1126/
science.abe9403
[35] Chen Z, Wang C, Feng X, et al. Interactomes of SARS-CoV-2 and human coronaviruses
reveal host factors potentially affecting pathogenesis. EMBO J. 2021;40:e107776.
doi:10.15252/embj.2021107776
[36] Kilkenny ML, Veale CE, Guppy A, et al. Structural basis for the interaction of SARS-CoV-
2 virulence factor nsp1 with DNA polymerase α–primase. Protein Sci. 2022;31:333–344.
doi:10.1002/pro.4220
[37] Borsatto A, Akkad O, Galdadas I, et al. Revealing druggable cryptic pockets in the
Nsp1 of SARS-CoV-2 and other β-coronaviruses by simulations and crystallography. eLife.
2022;11:e81167. doi:10.7554/eLife.81167
[38] Züst R, Cervantes-Barragán L, Kuri T, et al. Coronavirus non-structural protein 1 is a major
pathogenicity factor: implications for the rational design of coronavirus vaccines. PLoS Pathog.
2007;3:e109. doi:10.1371/journal.ppat.0030109
[39] Hojyo S, Uchida M, Tanaka K, et al. How COVID-19 induces cytokine storm with high
mortality. Inflamm Regen. 2020;40:37. doi:10.1186/s41232-020-00146-3
[40] Zhao K, Ke Z, Hu H, et al. Structural basis and function of the N terminus of SARS-CoV-2
nonstructural protein 1. Microbiol Spectr. 2021;9:e00169-21.
[41] Semper C, Watanabe N, Savchenko A. Structural characterization of nonstructural protein 1
from SARS-CoV-2. iScience. 2021;24:101903. doi:10.1016/j.isci.2020.101903
[42] Ma S, Mykhaylyk V, Bowler MW, et al. High-confidence placement of fragments into electron
density using anomalous diffraction – a case study using hits targeting SARS-CoV-2
non-structural protein 1. Int J Mol Sci. 2023;24(13):11197. doi:10.3390/ijms241311197